Understanding the Wavelength of Spectrophotometers
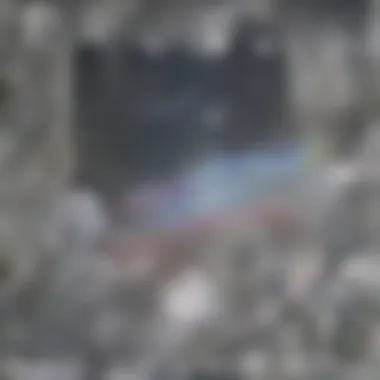
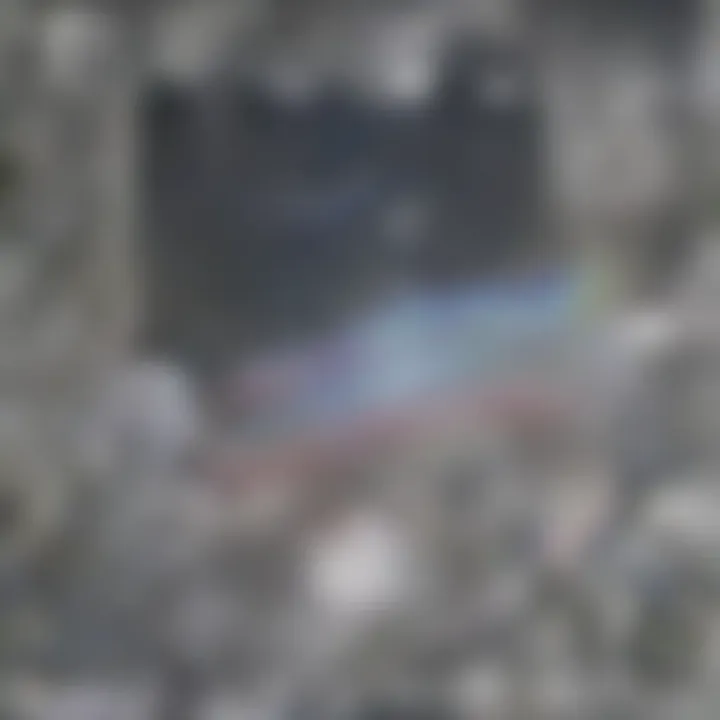
Intro
Wavelength plays a pivotal role in the operation of spectrophotometers. These devices are essential for measuring light absorption, transmission, and reflectance across various substances. Understanding wavelength allows researchers and industry professionals to optimize measurements and enhance accuracy in their analyses. This section will introduce the relevance of wavelength in spectrophotometry and its broader implications within scientific research.
Research Overview
Summary of Key Findings
In spectrophotometry, the wavelength of light directly influences the information obtained from samples. Through precise measurements at specific wavelengths, users can
- Identify chemical compounds
- Determine concentrations of substances
- Monitor changes in chemical reactions
One critical finding is that incorrect wavelength selection can lead to significant variations in results. This emphasizes the importance of calibrating spectrophotometers according to the intended application.
Methodologies Employed
The primary methodologies used in analyzing wavelength involve both theoretical calculations and practical testing.
- Theoretical Analysis: This includes mathematical modeling of light-matter interactions. It helps in understanding how different wavelengths behave when interacting with various compounds.
- Practical Calibration: Empirical methods ensure the spectrophotometer is tuned to the appropriate wavelengths necessary for specific experiments. Calibration against known standards is a common practice to maintain accuracy across various measurements.
In-Depth Analysis
Detailed Examination of Results
The results gathered from spectrophotometric measurements can reveal intricate details about sample composition. Different wavelengths may highlight specific properties due to their unique interactions with molecules. For example, ultraviolet light tends to be absorbed by bonds in organic compounds, while visible light can reflect more substantial structural features.
Comparison with Previous Studies
A comparative analysis of current research with historical studies demonstrates a trend toward improved wavelength precision. Earlier studies relied heavily on broad wavelength ranges, often missing crucial data points. Recent advancements now allow for narrower and more specific wavelength applications. This shift improves the finer details of chemical analysis and opens up new avenues for research across multiple fields.
"The precision in selecting the appropriate wavelength can either validate or invalidate an experimental hypothesis."
Prologue to Spectrophotometry
Spectrophotometry is a fundamental analytical technique widely used in various scientific fields. It enables researchers and professionals to determine the concentration of substances by measuring the intensity of light at particular wavelengths. Understanding the principles of spectrophotometry is crucial because it underpins many experimental methods used in research and industry. This section highlights the significance of spectrophotometry, its role in advancing scientific knowledge, and its application across disciplines.
The ability to measure absorbance or transmittance of light enables quantification of chemical compounds, biological substances, and environmental samples. This provides essential data that could guide critical decisions in research, diagnostics, and regulatory compliance. Particularly, the concept of wavelength is central to this process. When light interacts with matter, the resulting absorption spectra provide insight into the composition and behavior of different substances.
It is also important to consider that the accurate selection of wavelengths can influence measurement reliability. Thus, deeper knowledge of how spectrophotometers work and the factors affecting their function becomes imperative.
Definition of Spectrophotometry
Spectrophotometry is the study of how light interacts with matter, specifically focusing on measuring the intensity of light absorbed or transmitted through a sample. The core essence of this method lies in a device called a spectrophotometer. This instrument compares the amount of light entering a sample with the light emerging from it. In doing so, it quantifies the optical properties of the sample.
The relationship is typically defined using Beer-Lambert Law, where absorbance is directly proportional to the concentration of the absorbing species, the path length of light through the sample, and the molar absorptivity. This law serves as a foundation upon which spectrophotometric analysis is built, making it essential for precise measurements in laboratories.
Historical Development
The development of spectrophotometry can be traced back to advancements in optics and instrumentation. Early spectrophotometric methods emerged in the 19th century when scientists were beginning to understand the electromagnetic spectrum. Developments in prism technology allowed light to be dispersed into its constituent wavelengths, paving the way for measuring specific wavelengths of light.
The introduction of electronic detectors in the 20th century revolutionized the field, enhancing sensitivity and accuracy. As technology progressed, spectrophotometers evolved from simple devices measuring visible light, to sophisticated instruments capable of analyzing ultraviolet and infrared wavelengths. This evolution enabled applications in chemistry, biology, and environmental science, demonstrating the versatility and importance of spectrophotometry in modern research.
Wavelength: An Overview
Wavelength serves as a fundamental aspect of spectrophotometry. Understanding its role helps clarify how different wavelengths interact with materials, thus influencing measurement outcomes. The relationship between wavelength and energy levels is essential in any analytical technique that relies on the absorption, reflection, or transmission of light. By grasping these principles, users can optimize instrument settings and enhance the reliability of results.
Different wavelengths correspond to varying energies of electromagnetic radiation. This variability directly impacts how substances interact with light. Different materials absorb or transmit specific wavelengths, creating a unique spectral signature. Therefore, having a comprehensive understanding of the electromagnetic spectrum becomes crucial.
Wavelengthโs significance extends beyond mere measurement; it is integral during the selection of spectrophotometric techniques and instruments according to the analytical goal. Considerations around wavelength also span the operational conditions, such as environmental factors and sample composition.
"Wavelength not only defines the energy of light but also reveals the material characteristics that may remain hidden without precise spectral analysis."
With these points in consideration, the subsequent sections will delve deeper into the fundamental concepts behind the electromagnetic spectrum and the notable characteristics associated with wavelength.
Electromagnetic Spectrum
The electromagnetic spectrum encompasses all possible wavelengths of electromagnetic radiation. Ranging from radio waves at the lower end to gamma rays at the higher end, each segment of the spectrum has distinct properties and applications. Wavelengths associated with visible light range from approximately 380 nm to 750 nm. This segment is particularly relevant to spectrophotometry, where it is used for analyzing substances that interact with visible light.
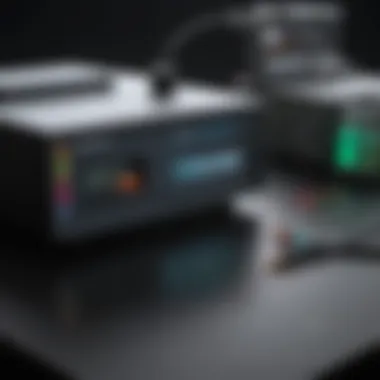
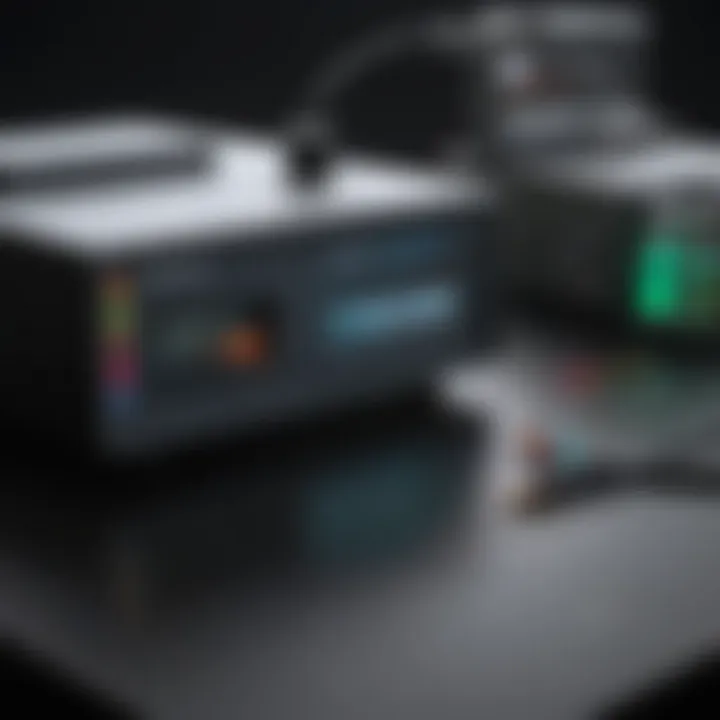
Within the electromagnetic spectrum, several key features are essential when applying spectrophotometry:
- Varied Wavelengths: Each segment of the spectrum corresponds to specific types of radiation, affecting measurement methods.
- Material Absorption: Different materials display unique absorption patterns across the spectrum, affecting data interpretation.
- Energy Transition: Changes in wavelength can indicate energy transitions within atoms and molecules, critical for analytical applications.
Understanding these elements is key for utilizing spectrophotometers effectively, as it enables researchers to select the appropriate measurement techniques tailored to their specific requirements.
Characteristics of Wavelength
The key characteristics of wavelength play a significant role in spectrophotometric measurements. The most notable ones include:
- Wavelength Measurement: Typically measured in nanometers (nm), wavelengths determine the extent of energy absorbed by materials.
- Spectral Resolution: This refers to the ability to distinguish between closely spaced spectral features. Higher resolution leads to better discernment of material characteristics.
- Intensity Variations: The intensity of light associated with a specific wavelength can vary, influencing the analysis of concentration in samples.
- Phase Changes: Wavelengths can denote phase changes in light, affecting interaction with samples and overall measurements.
Implementing the correct wavelength during analysis underpins various disciplines, such as biochemistry and environmental science. It is important to grasp the nuances of these characteristics to extract precise and meaningful data from spectrophotometric analyses.
The Science of Wavelength in Spectrophotometry
Understanding the science of wavelength in spectrophotometry is crucial. It directly impacts the accuracy and reliability of measurements in various scientific fields. The wavelength of light plays a significant role in how light interacts with matter, influencing the results obtained from spectrophotometric analysis. This section provides essential insights into this relationship and its implications for research and practical applications.
Relationship Between Wavelength and Energy
The concept of wavelength is inherently linked to energy. Light can be considered a form of energy that can be quantified. The energy of a photon is inversely proportional to its wavelength. In mathematical terms, the relationship can be articulated using the equation:
E = hc/ฮป
Where:
E = energy of the photon,
h = Planck's constant,
c = speed of light, and
ฮป = wavelength of the light.
Shorter wavelengths correspond to higher energy photons. Hence, ultraviolet light has more energy than visible light, and visible light has more energy than infrared light. This characteristic is vital in spectrophotometry. When substances absorb light, they typically do so at specific wavelengths correlating with their energy states. Understanding this relationship allows scientists to select appropriate wavelengths for measuring different compounds or materials.
The key points include:
- Higher energy means a shorter wavelength.
- Different substances absorb light differently based on their molecular structures.
- The right wavelength can lead to more accurate measurements.
Interaction of Light with Matter
When light encounters a sample in a spectrophotometer, its interaction can lead to various outcomes: absorption, transmission, and scattering. The way a particular substance interacts with different wavelengths of light provides essential information about its chemical composition, concentration, and other properties.
Absorption occurs when the energy of the photon matches the energy required for electrons in atoms or molecules to jump to higher energy levels. This phenomenon forms the basis of how spectrophotometry measures concentration. By assessing the amount of light absorbed at various wavelengths, researchers can infer the concentration of compounds in a solution.
There are several crucial points to consider regarding the interaction of light with matter:
- Absorption Spectroscopy: This technique relies on the selective absorption of light at specific wavelengths to identify and quantify substances.
- Beer's Law: This law states that the absorbance is directly proportional to the concentration of the absorbing species in the solution, allowing for quantitative analysis.
- Transmission and Scattering: These interactions, while important, can introduce complications and potential errors in measurements, thus necessitating careful calibration and validation of the spectrophotometer settings.
In summary, understanding both the relationship of wavelength with energy and the interaction of light with matter is fundamental in spectrophotometry. These concepts provide the foundation for effective analysis and accurate results across a wide range of applications in science.
Types of Spectrophotometers
Spectrophotometers are essential tools for scientists and researchers across various fields. Understanding the different types of spectrophotometers is vital for selecting the right equipment that aligns with specific experimental needs. This section explores the main types, highlighting their unique features, advantages, and practical considerations.
Visible Spectrophotometers
Visible spectrophotometers measure the absorbance or transmittance of light in the visible spectrum, typically ranging from 400 to 700 nm. These instruments are widely utilized in laboratories for applications such as colorimetric analysis, where the concentration of colored solutions is established by measuring the intensity of light absorbed. Visible spectrophotometers are often simpler and more cost-effective than their UV counterparts, making them accessible for routine analysis.
Key features include:
- Broad Stability: They provide stable measurements due to standardized light sources such as tungsten or halogen bulbs.
- User-Friendly: Most models are designed with intuitive interfaces, making them suitable for users at different skill levels.
- Versatile Applications: Commonly used in biochemistry, environmental monitoring, and food quality control.
However, it is important to consider that visible spectrophotometers may have limitations regarding the detection of highly concentrated solutions, which may require dilution prior to measurement.
UV-Visible Spectrophotometers
UV-visible spectrophotometers expand the range of measurement to include both ultraviolet and visible wavelengths, typically from 200 to 800 nm. This allows for the analysis of various compounds, including nucleic acids and proteins, which absorb strongly in the UV region. UV-visible spectrophotometers are indispensable in molecular biology laboratories, offering enhanced sensitivity compared to visible-only instruments.
Advantages include:
- Enhanced Sensitivity: They can detect lower concentrations of substances based on their absorption characteristics in the UV region.
- Broader Range of Applications: They are used in pharmaceutical analysis, quality control, and forensic science.
- Real-Time Monitoring: Some models allow for kinetic studies by recording changes in absorbance over time, providing valuable insights into reaction mechanisms.
While UV-visible spectrophotometers are powerful analytical tools, users must take precautions against issues such as stray light and instrument drift, which can affect accuracy.
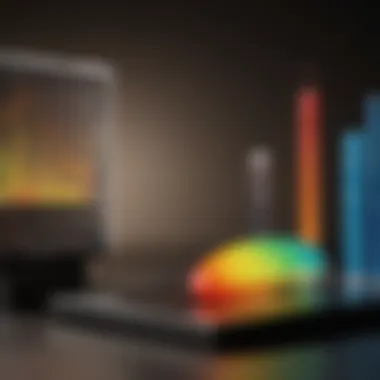
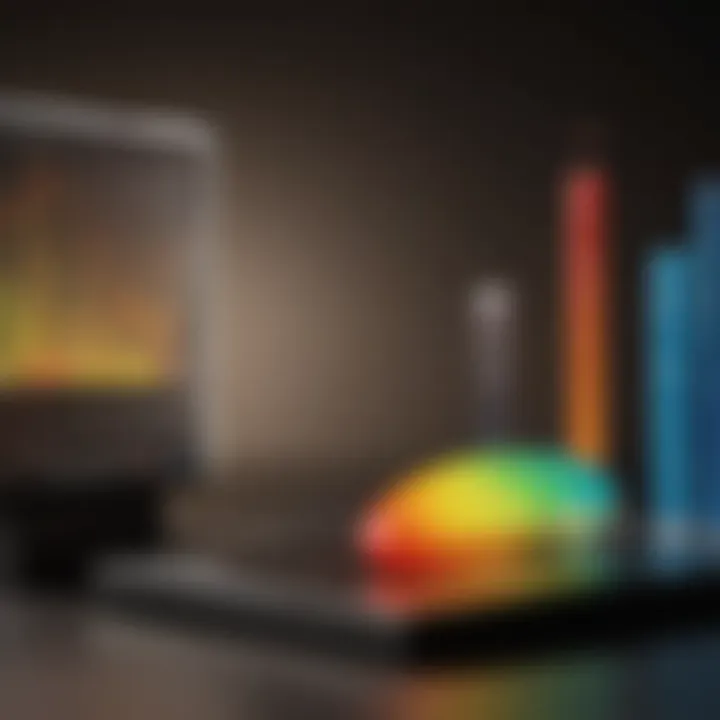
Infrared Spectrophotometers
Infrared spectrophotometers operate in the infrared region of the electromagnetic spectrum, typically from 700 nm up to 1 mm. These instruments are particularly useful for identifying molecular structures and functional groups in organic compounds. The technique is commonly employed in industries such as petrochemicals, pharmaceuticals, and environmental assessments.
Notable features include:
- Molecular Fingerprinting: They provide unique spectral patterns that can be used for compound identification.
- Non-Destructive Analysis: Infrared spectroscopy is often non-destructive, allowing for the analysis of samples without altering their physical state.
- Advanced Techniques: Instruments may include techniques such as Fourier-transform infrared (FTIR) spectroscopy, which significantly enhance resolution and sensitivity.
Nevertheless, it is important to ensure proper sample preparation, as the presence of moisture and contaminants can interfere with readings.
"Choosing the right type of spectrophotometer directly influences the accuracy and reliability of analytical results. It is essential to understand each type's capabilities to meet specific research and application needs."
Principles of Wavelength Selection
Wavelength selection is a fundamental aspect in spectrophotometry. Accurate measurement relies on using the correct wavelength for a specific application. This section discusses the importance of monochromatic light sources and the role of filters and gratings in selecting wavelengths. Understanding these principles enhances the reliability of spectroscopic analysis in diverse scientific fields.
Monochromatic Light Sources
Monochromatic light sources emit light of a single wavelength. Using such sources improves measurement precision since it minimizes noise and interference from other wavelengths. Common monochromatic light sources include:
- Lasers: These offer high coherence and stability at a single wavelength, making them ideal for analytical applications.
- Tungsten Lamps: Suitable for visible light applications but emit broad spectra, thus often paired with filters for wavelength control.
- Deuterium Lamps: Used primarily in UV spectrophotometry, they provide a continuous spectrum but need additional devices to isolate desired wavelengths.
Choosing the right light source is crucial because the application's inherent requirements influence accuracy. The emitted wavelength must match the absorption characteristics of the analyte being measured, ensuring accurate quantification.
Filters and Gratings
Filters and gratings are employed to isolate specific wavelengths from a broader spectrum. They serve to enhance measurement reliability by ensuring that only the desired wavelengths reach the sample. There are two principal types:
- Optical Filters: These include bandpass, long pass, and short pass filters. Bandpass filters allow a specific range of wavelengths to pass while blocking others. This selective filtration is essential in eliminating background interference during analysis.
- Diffraction Gratings: These devices disperse light into its component wavelengths using physical principles. They are versatile and can separate closely spaced wavelengths, allowing for high-resolution measurements. Gratings are crucial in advanced spectrophotometric systems, enabling comprehensive spectral analysis.
By understanding how to select the appropriate wavelength sources and isolation techniques, researchers can improve the accuracy of their results significantly. Each method has unique advantages depending on the specific requirements of the analysis.
"Precision in wavelength selection directly correlates with the reliability of spectrophotometric measurements."
Incorporating these principles leads to enhanced analytical capability, vital for ongoing research and applications in various scientific domains.
Calibration and Validation of Spectrophotometers
Calibration and validation of spectrophotometers represent crucial processes ensuring measurement accuracy and reliability in scientific research. These practices establish a framework for verifying that the instrument functions within defined limits, responding consistently to the correct standards. This section delves into the significance of calibration and the methods used to achieve effective validation.
Importance of Calibration
Calibration is vital for maintaining the integrity of spectrophotometric measurements. It involves adjusting and verifying the instrument's parameters against known standards. Without rigorous calibration, the data obtained from experiments can become misleading or incorrect, potentially leading to erroneous conclusions in research or industrial applications.
The benefits of systematic calibration include:
- Consistency in Results: Regular calibration reduces variances in measurements, helping ensure that data can be replicated.
- Enhanced Accuracy: It aligns the instrument readings with established values, minimizing systematic errors that may arise from misinterpretation of spectra.
- Regulatory Compliance: For industries such as pharmaceuticals and environmental testing, adherence to strict regulatory standards necessitates well-calibrated equipment.
- Quality Control: Calibration acts as a quality assurance mechanism, providing confidence in the results reported to stakeholders.
Methods of Calibration
Different methods exist for calibrating spectrophotometers, each suitable for various applications and types of instruments. The most common approaches include:
- Standard Solution Calibration: This method involves measuring the absorbance of a set of standard solutions with known concentrations. The absorbance readings are then plotted against concentration to derive a calibration curve. This is fundamental in quantitative analysis.
- Wavelength Calibration: Ensuring that the instrument accurately measures the wavelength of incident light is essential. This is often done using spectral lamps with narrow emission lines, such as mercury or sodium lamps. The instrument's readings are checked against these known emission lines.
- Factory Calibration: Many spectrophotometers are calibrated at the factory before delivery. Users should periodically verify this calibration to ensure ongoing accuracy.
- Software-Based Calibration: Modern spectrophotometers often include software that can assist in calibration processes. Advanced algorithms and data analysis tools can help tailor calibrations for specific applications more rapidly.
Applications of Wavelength in Scientific Research
The application of wavelength in scientific research stands as a foundational element across various disciplines. Understanding how light interacts with materials allows researchers to derive meaningful insights and make informed decisions. It provides an essential framework for estimating concentration levels, identifying materials, and investigating dynamic processes. In this context, precise wavelength selection is critical for ensuring accuracy in results across biochemical analysis, environmental monitoring, and industrial applications.
Biochemical Analysis
Biochemical analysis relies heavily on wavelength measurement for a range of assays and validations. For instance, ultraviolet-visible (UV-Vis) spectrophotometry is commonly used to assess the concentration of biomolecules like proteins and nucleic acids. Different compounds absorb light at specific wavelengths, creating unique absorption spectra. By measuring absorbance at those wavelengths, researchers can quantify sample concentrations with remarkable precision.
In practice, each biomolecule has characteristic absorption peaks. For example, nucleic acids show strong absorbance at 260 nm. This correlation allows researchers to determine the purity and concentration of DNA or RNA by measuring absorbance ratios. Accurate wavelength measurement leads to reliable outcomes, enhancing experimental reproducibility across the field.
Environmental Monitoring
Wavelength applications also extend to environmental monitoring, where the analysis of light absorption and transmission provides crucial data about pollutants and natural resources. For instance, monitoring water quality often involves measuring absorbance to detect contaminants. Specific wavelengths can indicate the presence of substances such as nitrogen compounds, heavy metals, or organic pollutants. This approach empowers researchers and policymakers to implement strategies for pollution control and environmental protection.
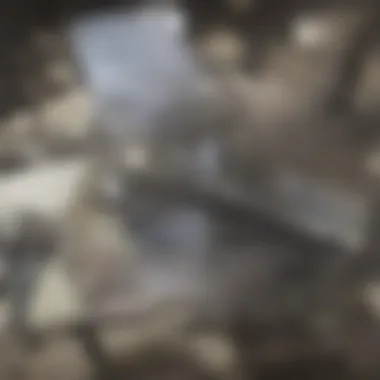
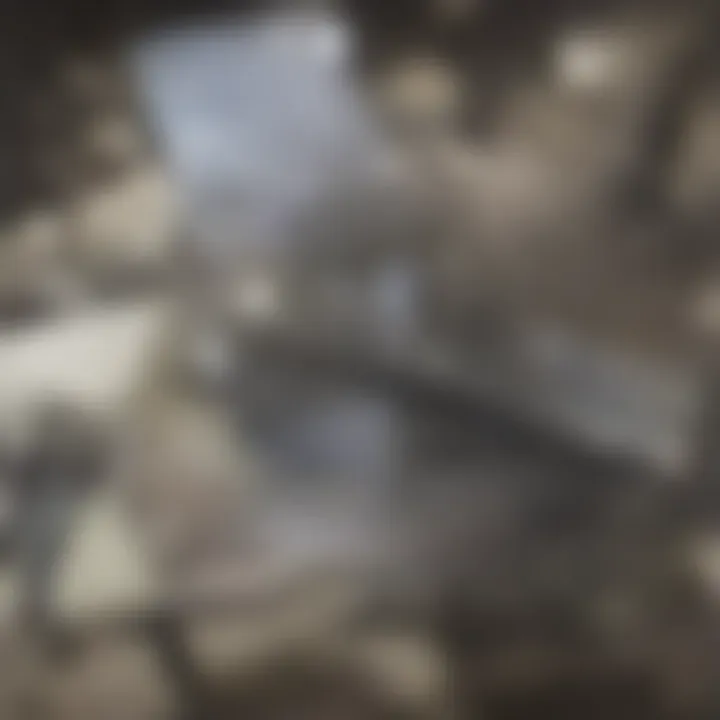
Additionally, satellite remote sensing utilizes wavelength data to evaluate land cover, vegetation health, and atmospheric conditions. By understanding how different materials reflect or absorb light at varying wavelengths, scientists can gather information about ecosystem changes, climate variations, and habitat loss. This knowledge is vital for addressing environmental challenges in a systematic manner.
Industrial Applications
The industrial sector leverages wavelength applications to improve product quality, safety, and efficiency. For example, spectrophotometry is a vital tool in food and beverage production. It can be employed to monitor color and clarity during manufacturing processes, thereby ensuring that products meet defined quality standards. By optimizing the control of light wavelength, companies can produce consistent products, reinforcing consumer trust.
Moreover, in pharmaceuticals, wavelength-specific assays are crucial for drug formulation and quality control. Validating active ingredients based on their absorbance properties allows companies to maintain regulatory compliance and safeguard consumer health. In manufacturing environments, using wavelength analysis can enhance efficiency by enabling real-time monitoring of processes.
In summary, the applications of wavelength in scientific research are both extensive and vital. Each fieldโbiochemical analysis, environmental monitoring, and industrial applicationsโbenefits significantly from an understanding of how wavelength influences measurement accuracy and methodology. Future developments in wavelength technology and analysis will likely lead to even greater advancements in research and application.
Challenges and Limitations in Wavelength Measurement
Accurate wavelength measurement is essential in spectrophotometry. It dictates the reliability of data and the validity of subsequent analysis. Despite this importance, challenges and limitations often arise in measuring wavelengths. Understanding these challenges allows scientists to develop better methodologies and refine their techniques. This section explores the sources of error and how to resolve issues in wavelength measurement.
Sources of Error
When measuring wavelength with a spectrophotometer, various sources of error can impact the results. Some common ones include:
- Instrument Calibration: If the spectrophotometer is not calibrated correctly, it may register wavelengths inaccurately. This miscalibration can skew results.
- Environmental Factors: Ambient light, temperature, and humidity can influence measurements. Variations in these conditions may lead to inconsistent wavelength results.
- Sample Preparation: The way samples are prepared affects the light's interaction with matter. Impurities or improper concentrations can cause misleading readings.
- Detector Limitations: Each detector type has its sensitivity range. Using a detector not suited for the specific wavelength can result in poor readings.
Accurate calibration and careful sample handling are critical for minimizing error in wavelength measurements.
Understanding these potential pitfalls is crucial for anyone working with spectrophotometers. Identifying errors in their infancy is important to prevent larger issues in data interpretation.
Resolving Measurement Issues
Addressing measurement issues involves several strategies to enhance wavelength measurement reliability:
- Regular Calibration: Frequent calibration of instruments ensures they remain within their specified parameters. Follow manufacturer guidelines for calibration intervals.
- Controlled Environment: Conduct measurements in a stable environment, reducing external variables. Use dedicated rooms with controlled temperature and humidity if possible.
- Standard Operating Procedures: Establish standardized protocols for sample preparation. This consistency aids in minimizing variations in results.
- Data Validation: Always validate results with controls or standard solutions. This practice provides reference points for accuracy.
By adopting these strategies, researchers can enhance the accuracy of their wavelength measurements. Addressing challenges head-on is paramount for advancing reliable spectrophotometric applications. Proper attention to these issues ultimately contributes to the robustness of scientific research.
Future Trends in Spectrophotometry
Spectrophotometry is an evolving field, and the future holds significant promise for advancements in technology and application. Understanding these trends is critical as they will define how spectrophotometers are utilized in scientific and industrial settings. Enhanced measurement capabilities, efficiency, and integration into broader analytical frameworks are just some of the areas where we see change. As the demand for precise and versatile analytical tools continues to increase, spectrophotometers will need to adapt accordingly.
Technological Advancements
Technological advancements in spectrophotometry are pushing the boundaries of what these instruments can do. A major focus is on improving the sensitivity and resolution of measurements. For example, developments in detector technology have led to the creation of more responsive systems that can detect lower concentrations of substances. This is particularly important in fields like biochemical analysis, where minute changes can be vital for accurate assessments.
Additionally, advancements in software have made data analysis more intuitive. Modern spectrophotometers often come equipped with sophisticated algorithms that enhance data interpretation. This not only aids researchers in deriving accurate conclusions from complex data sets but also allows for real-time analysis during experiments.
Besides software improvements, the miniaturization of spectrophotometers is notable. Compact devices that can deliver high-quality data are particularly useful in fieldwork and remote analysis. They enable researchers to perform experiments outside of traditional laboratories, increasing their versatility.
Integration with Other Analytical Techniques
Integration of spectrophotometry with other analytical techniques is another significant trend. As scientific inquiries become more interdisciplinary, the need for instruments that can work seamlessly with other methods is essential. For instance, combining spectrophotometry with chromatography allows for more comprehensive analysis of chemical compositions.
When spectrophotometry is coupled with techniques such as mass spectrometry or nuclear magnetic resonance, researchers gain multifaceted insights that are not possible with single-method approaches. This integration can enhance the accuracy of measurements by providing complementary data that confirms findings across different methodologies.
Furthermore, the rise of multi-modal analytical systems presents new opportunities. Researchers can now conduct simultaneous analyses using various methods, which significantly accelerates the workflow and enriches the data pool.
In summary, these trends highlight that future spectrophotometers will not only be more refined in their measurement capabilities but also versatile in their application. Awareness and adaptation to these changes will be essential for professionals in fields that rely on precise spectral data.
Closure
The conclusion section serves as a pivotal element in this article on spectrophotometry and the significance of wavelength. It encapsulates key lessons learned while reinforcing the foundational concepts discussed. Understanding the role of wavelength in spectrophotometry offers tremendous benefits across various scientific disciplines. It not only maximizes the accuracy of measurements but also enhances the design and calibration processes of spectrophotometers.
Summary of Key Points
- Definition of Wavelength: Wavelength is a critical parameter in determining the behavior of light and how it interacts with matter. This fundamental concept underpins all spectrophotometric activities.
- Relationship with Energy: The connection between wavelength and energy establishes essential principles for excitation and absorption in various materials.
- Application Across Disciplines: The utilization of wavelength extends to biochemical analysis, environmental monitoring, and various industrial applications, showcasing its versatility.
- Technological Advancements: Innovations in spectrophotometric technologies enhance accuracy and integrate methodologies with other analytical techniques, leading to improved outcomes in research and industry.
In summary, the understanding of wavelength is integral for those working with spectrophotometers, encouraging precise applications and refined methods.
Implications for Future Research
Future research inspired by the insights gained from this article can shift focus toward several promising areas:
- Advanced Calibration Techniques: As precision demands increase in scientific measurement, developing more sophisticated calibration methods could enhance measurement accuracy significantly.
- Integration with Other Technologies: The potential for integrating spectrophotometry with technologies like machine learning or data analytics presents new opportunities for innovative applications.
- Exploration of New Wavelength Ranges: Investigating less commonly used regions of the electromagnetic spectrum may unveil new applications in varied fields such as medicine, environmental science, and materials development.
The ongoing evolution in the understanding of wavelength in spectrophotometry promises to advance scientific inquiry and practical applications significantly.
Overall, the conclusions drawn from this exploration lay a strong foundation for ongoing study and exploration, informing future advancements in the discipline.