The Role and Evolution of Competent Cells in Molecular Biology
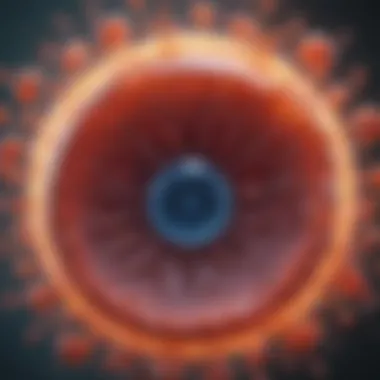
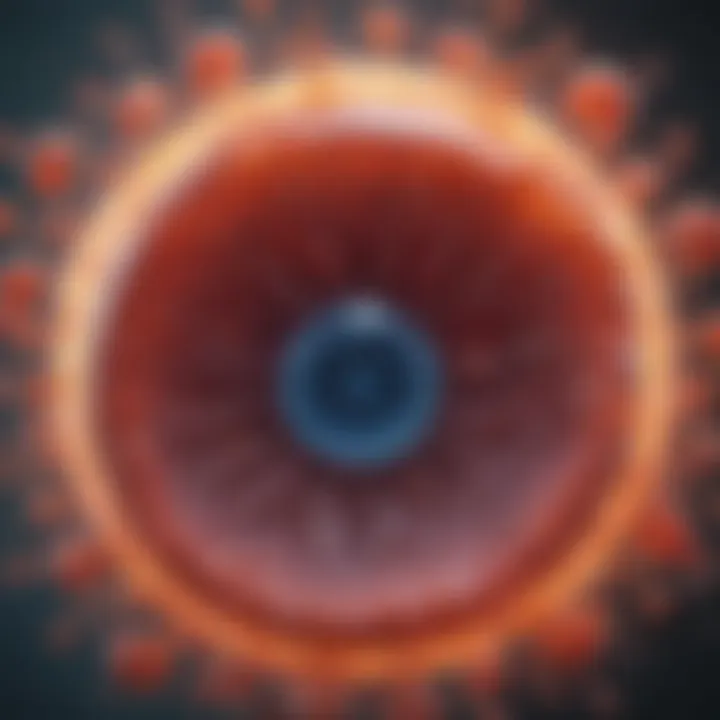
Intro
The world of molecular biology is filled with a myriad of techniques that play pivotal roles in advancing our understanding of life at the cellular level. Among these, the transformation of competent cells stands out as a cornerstone practice. This process is not just a technical procedure; it is a gateway to introducing foreign DNA into host cells, thus enabling researchers to manipulate genetic material for various applications. Think of it as handing over a set of blueprints from one engineer to another, allowing for an expansion of ideas, creativity, and ultimately, innovation in the realms of genetic engineering and recombinant protein production.
In the coming sections, we will unwrap the intricacies of cell competence and the methods used to create these responsive cells. We'll delve into how the science harnesses this capability to push the boundaries of research. Expect insights on contemporary advancements and some of the hurdles faced by scientists today.
Let’s embark on this exploration to understand not only the processes involved but also their significance in shaping the future of genetic manipulation.
Prelims to Competent Cells
In the realm of molecular biology, the significance of competent cells cannot be overstated. They serve as the gateway for introducing foreign DNA into host organisms, thus permitting a vast array of genetic modifications and research applications. Whether one is involved in cloning genetic sequences or producing recombinant proteins, understanding how these cells come to be and function is crucial for any biologist.
Definition and Importance
Competent cells are cells that have the innate ability or are induced to take up external DNA from their environment. This process is known as transformation. The importance of this property lies in several core areas:
- Genetic Engineering: Scientists manipulate genes to create organisms with desired traits.
- Biopharmaceutical Production: Competent cells often serve as factories for producing valuable proteins and enzymes, including insulin and monoclonal antibodies.
- Research Applications: They enable researchers to explore gene functions, interactions, and regulatory mechanisms.
In essence, they’re the unsung heroes of countless applications in genetic sciences, acting as the vessels of innovation.
Historical Context
The journey to understanding competent cells is marked by a rich tapestry of discoveries. The term ‘competence’ can trace its origins back to the 1920s when Frederick Griffith uncovered the phenomenon of transformation in bacteria. He demonstrated that non-virulent strains of Streptococcus pneumoniae could uptake DNA from heat-killed virulent strains, thus becoming pathogenic themselves. This groundbreaking finding laid the foundation for later molecular biology advancements.
Fast forward to the 1970s, when plasmids, small circular DNA molecules, became prevalent in genetic manipulation. Researchers sought to exploit competent cells to maximize plasmid uptake, leading to the development of chemical and physical methods for inducing competence. Key methodologies were established, such as the calcium chloride treatment and electroporation techniques, which remain widely used today.
"The evolution of competent cells reflects our own progress in the realm of genetic research—all built on the shoulders of giants who paved the way."
These historical milestones not only highlight the techniques but also underscore the driving curiosity that has defined molecular biology's trajectory. As new challenges and opportunities arise, the exploration into competent cell innovations continues to evolve, reflecting ongoing advancements in the science and technology aspects of genetic studies.
Understanding Cell Competence
Understanding cell competence is crucial for researchers and practitioners delving into molecular biology. Competence refers to a cell's ability to take up exogenous DNA from its surrounding environment. This characteristic is the linchpin for various genetic engineering applications, providing the tools necessary for cloning, protein production, and gene function analysis. The nature and mechanisms of cell competence can drastically influence experimental outcomes and, therefore, require a comprehensive study.
Natural Competence vs. Artificial Competence
Natural competence is a phenomenon observed in certain bacterial species—including Bacillus subtilis and Neisseria gonorrhoeae—where cells can naturally acquire DNA from their environment without any artificial tools or agents. These organisms possess complex receptor systems that facilitate the recognition and uptake of extracellular DNA. Natural competence is typically regulated in a manner closely tied to specific growth conditions, such as stress or nutrient depletion.
On the other hand, artificial competence is induced in laboratory settings and is often achieved by treating cells with chemical agents like calcium chloride or using electroporation techniques. This method increases the permeability of the cell membrane, allowing for the effective uptake of foreign DNA constructs. The choice between natural and artificial competence hinges on various factors, including the target organism, the type of DNA to be introduced, and the desired efficiency of transformation.
- Natural Competence:
- Artificial Competence:
- Occurs in some bacteria naturally.
- Depends on environmental conditions.
- Not as controllable as artificial methods.
- Induced via chemical treatments or electroporation.
- Offers researchers a reliable approach to transformation.
- Flexibility in selection of bacteria.
Molecular Mechanisms of Competence
The molecular mechanisms behind competence are intricate and fascinating. In naturally competent bacteria, competence genes are often tightly regulated by environmental signals. Various signaling pathways converge to activate the transcription of these competence genes, leading to the expression of proteins that facilitate DNA binding and uptake.
When it comes to artificial competence, the preparation of competent cells typically involves manipulating the cellular membrane's properties. For instance, in the calcium chloride method, treated cells gain temporary openings in their membranes, referred to as "pores," allowing for the passage of DNA. On another front, electroporation employs a brief electrical field to create lipid bilayer distortion, enabling DNA to enter the cell.
"Understanding the molecular pathways involved in competence not only enriches our grasp of microbial genetics but also opens doors to innovations in synthetic biology."
These mechanisms matter significantly because they determine the efficiency of DNA uptake and subsequent expression. Consequently, optimizing these processes to maximize efficiency is a focal point of ongoing research in genetic engineering. Pursuing this understanding can yield breakthroughs that refine how genetic material is introduced into cells, a critical step in applications ranging from gene therapy to vaccine development.
Methods for Preparing Competent Cells
The methods for preparing competent cells are critical in the field of molecular biology. These techniques not only enable the introduction of foreign DNA into host cells but also set the stage for various downstream applications like cloning and recombinant protein production. Understanding the nuances of each preparation method is tantamount to selecting the right approach for particular experiments. The benefits of these methods include enhanced transformation efficiencies, reproducibility, and adaptability to various types of DNA. However, each method comes with its own set of considerations that must be evaluated before application.
Chemical Methods
Chemical methods are among the most widely adopted ways to render cells competent, particularly in laboratory settings where controllable conditions are essential. These approaches tend to be straightforward and relatively cost-effective, allowing researchers to prepare competent cells quickly while maintaining high efficiency.
Calcium Chloride Treatment
Calcium chloride treatment is a classic method used to prepare competent cells. The process involves suspending bacterial cells in a calcium chloride solution, which allows the cells to become permeable to DNA. The primary characteristic that makes calcium chloride treatment popular is its ease of application and effectiveness.
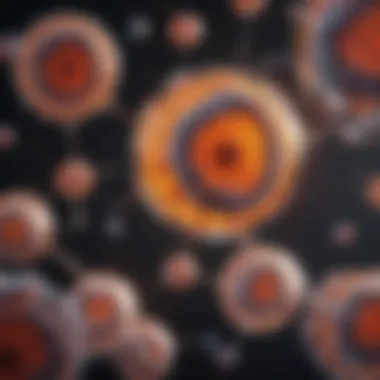
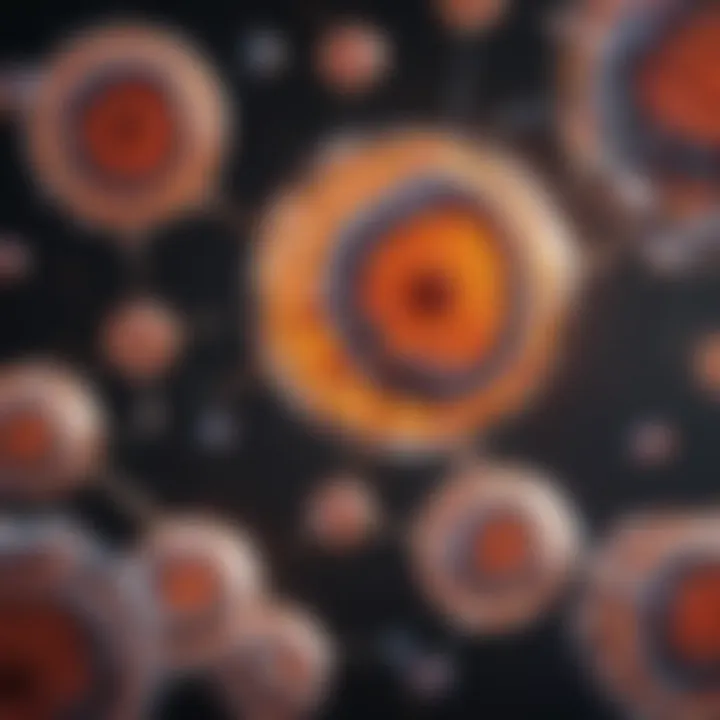
One unique feature of this method is how the high ionic strength of the calcium chloride solution can facilitate DNA uptake. This makes it a favored choice among many microbiology labs, especially those just getting their feet wet in molecular techniques.
However, the calcium chloride method does have its downsides. For instance, it can yield lower transformation efficiencies compared to some more sophisticated techniques. Also, the method requires precise handling, as over-dilution could lead to decreased competency. Still, when cost and simplicity are prioritized, calcium chloride treatment rises to the forefront.
PEG (Polyethylene Glycol) Method
The PEG method utilizes polyethylene glycol to enhance the uptake of DNA by competent cells. PEG effectively encourages the fusion of the cell membrane with the DNA, leading to an increase in transformation success rates. One key characteristic that sets PEG apart is its ability to outperform some chemical methods when it comes to transformation efficiency.
A unique advantage of using the PEG method is that it can be highly adaptable, allowing for the optimization of conditions based on specific experimental needs. This can be particularly helpful when working with large plasmids or when higher transformation rates are desired.
Nevertheless, the PEG method does have some drawbacks. The preparation may require more steps and materials than simpler chemical methods, resulting in longer preparation times. Additionally, PEG's concentration must be carefully monitored since too high a level can lead to cell lysis.
Electroporation Techniques
Electroporation is another potent technique for preparing competent cells, leveraging electric fields to facilitate DNA uptake. It allows for a wide range of DNA sizes to be introduced into cells, making it a versatile choice for researchers.
Principles of Electroporation
The principle behind electroporation lies in the application of short electrical pulses to a cell suspension. This sudden electric field creates temporary pores in the cell membrane, allowing DNA to be introduced. One of the key characteristics that make this method advantageous is its high efficiency, particularly for larger plasmids or even linear DNA.
Electroporation allows precise control over several parameters, such as pulse strength and duration, making it customizable to the needs of different experimental setups. Despite its benefits, the technique does require specialized equipment and can potentially lead to cell death if conditions are not optimized correctly.
Factors Influencing Electroporation Efficiency
The efficiency of electroporation can be affected by a multitude of factors, including cell type, electrical field strength, and pulse duration. Each of these elements must be delicately balanced to achieve optimal results. A defining characteristic of this method is how the condition of the cells prior to electroporation—such as growth phase—can significantly influence outcomes.
One unique benefit here is that optimizing these factors can lead to a dramatic increase in transformation efficiency, making it a valuable choice for high-stakes experiments. However, the need for precise adjustments means that researchers must be diligent and methodical in their approach.
In molecular biology, the choice of competent cell preparation method can have significant implications for experimental outcomes, determining not only efficiency but also the success of subsequent applications.
Transformation Process Overview
Understanding the transformation process is crucial in molecular biology, as it lays the foundation for many applications, from cloning genes to producing proteins. This process refers to the introduction of foreign DNA into competent cells, enabling researchers to manipulate genetic material efficiently. Transformations are not merely essential steps; they represent gateways to innovation in genetic research and biotechnology.
The importance of transformation lies in its versatility and adaptability to various methods and approaches. A well-optimized transformation protocol can increase the success rate significantly, allowing even minute quantities of DNA to yield robust results. Moreover, the methods of introduction and subsequent recovery are intricate, comprising several critical factors that can make or break an experiment.
Preamble of DNA
The step of introducing DNA into competent cells is where the magic actually happens. Whether using a chemical method or electroporation techniques, this act serves as the bridge between foreign material and host cell. The DNA that researchers introduce may come from a range of sources, including plasmids, PCR products, or even genomic DNA.
- Chemically Induced Transformation: In methods like the calcium chloride treatment, cells are exposed to a solution containing calcium ions, which helps the DNA cross the cell membrane. After a short incubation, the cells take up the DNA, an event that's dictated by several environmental variables and the condition of the cells.
- Electroporation: Alternatively, electroporation employs an electric pulse to create temporary pores in the cell membrane, allowing DNA to slip in more freely. This technique has gained popularity due to its efficiency and ability to handle different types of DNA.
Each method possesses unique features and challenges. The choice often depends on the strategic goals of the experiment and the materials at hand.
Post-Transformation Recovery
Once DNA has been introduced, a critical recovery phase begins. This stage is pivotal, as it determines whether the transformed cells can express the new genetic material. Typically, cells are incubated in a suitable recovery medium that allows them to stabilize and begin expressing any newly introduced genes and proteins.
During recovery:
- Nutrient Absorption: The cells are provided with nutrients that aid in cellular repair and growth, which can contribute to better transformation efficiency. Nutrient media are crafted to replenish the energy that cells lose during the transformation process.
- Time Matters: Too short a recovery time may lead to insufficient expression, while too long can create complications like cell overgrowth or senescence.
- Boosting Efficiency: Some researchers may choose to add selective agents to the recovery medium to enhance transformation rates or ensure only successfully transformed cells survive.
According to recent findings, the recovery phase is as important as the transformation step itself, and neglecting either can lead to low yields of transformants. Heeding the nuances of this process presents opportunities to enhance efficiency and achieve desired outcomes in genetic experiments.
"In molecular biology, overlooking the intricacies of transformation can be the difference between success and failure. Every detail counts."
In summary, the transformation process serves as a critical pathway to achieve the goals of molecular biology. From the introduction of DNA to the essential recovery stage, understanding the complexities involved is vital for researchers seeking to explore the frontiers of genetic manipulation.
Factors Affecting Transformation Efficiency
The efficiency of transforming competent cells is a critical factor in molecular biology. Essentially, this efficiency indicates how well foreign DNA can be introduced into a host cell. High transformation efficiency ensures more successful experiments, making it easier to achieve the desired genetic manipulation outcomes. Several elements come into play when assessing transformation efficiency, and a proper understanding of these can lead researchers to refine their processes to achieve better results.
Cell Growth Phase and Condition
One of the most influential factors affecting transformation efficiency is the growth phase of the competent cells. Harvesting cells at the right time is crucial. For instance, cells in the mid-log phase tend to take up DNA more effectively than those in stationary or lag phases. During the mid-log phase, cells are actively dividing and have optimal membrane permeability, allowing for enhanced DNA uptake.
Inadequate growth conditions also negatively affect transformation. Cells must be handled gently and should not undergo harsh treatments before transformation. It's vital to keep them in a nutrient-rich media, which sustains their growth while reducing stressors that could impede efficiency. In summary, keeping a close watch on cell growth and their overall condition before transformation can mean the difference between success and failure in experimental pursuits.
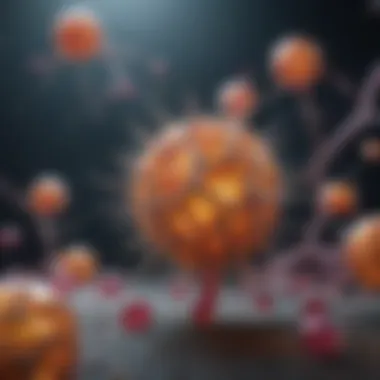
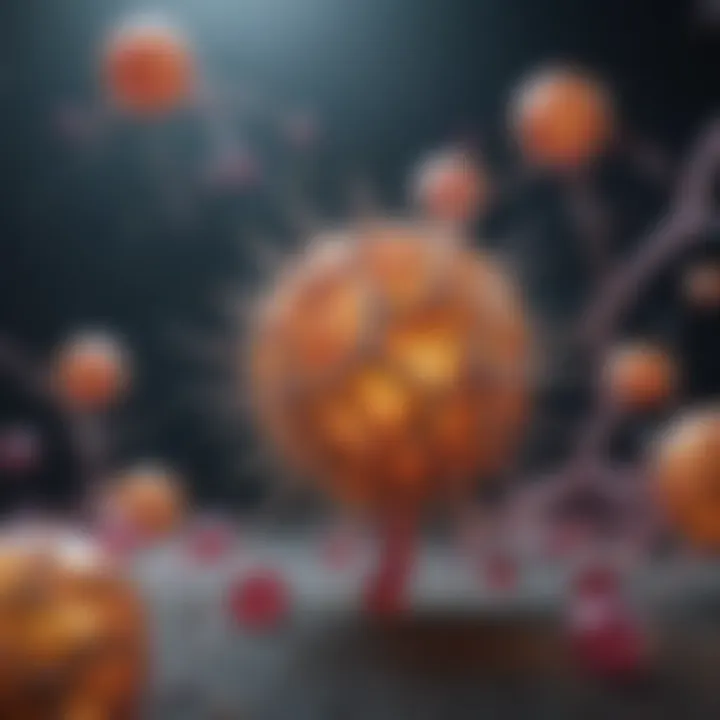
DNA Quality and Quantity
The quality and quantity of DNA used for transformation hold equal significance. Poor quality DNA, which may come from degradation or contamination, can drastically reduce the transformation rates. On the other hand, high-quality, purified plasmid DNA is ideal as it leads to a more effective integration into the competent cells.
Additionally, the amount of DNA is another vital consideration. If there’s too little DNA, the chances of transformation drop; conversely, using excessive amounts does not necessarily lead to improved outcomes and may even inhibit the process. Optimization is often required, striking a balance to ensure that the quantity of DNA is sufficient but not excessive. The recommendation is generally to use a concentration that reflects the rough estimates of what works well in various protocols—typically in the range of 50 to 100 nanograms per microliter.
Environmental Factors
Temperature
Temperature presents a pivotal aspect in transformation efficiency. Research indicates that cooling the cells and DNA solution can promote DNA uptake. For example, maintaining a temperature around 0 degrees Celsius during the incubation of competent cells with DNA has been shown to improve transformation rates. Doing so helps protect DNA from nucleases that might otherwise degrade it. Furthermore, the shift to a warmer temperature during the heat shock step enhances membrane fluidity, thus allowing easier integration of the genetic material.
However, one must exercise caution; maintaining excessively cold temperatures can hinder cellular functions and compromise cell integrity. The challenge lies in finding that sweet spot where the temperature, while cold enough to protect DNA, doesn’t impede cell health.
Salinity
Salinity is another environmental factor that merits attention. The ionic conditions of the solution in which competent cells are incubated can affect transformation efficiency. Cells are commonly exposed to a salt solution (like calcium chloride) during preparation because it helps stabilize their membranes and facilitates DNA binding.
However, one must consider that overly high salinity can have an adverse effect. It might lead to cell lysis or hinder the uptake of DNA by creating an overly viscous environment. The key is moderation—adjusting salt concentration to optimum levels can support transformation without causing detrimental effects to the cells themselves. Therefore, knowing the right salinity can prove beneficial in optimizing transformation processes.
Applications of Competent Cell Transformation
The role of competent cell transformation is essential in modern molecular biology. Researchers have leveraged this technology to push the boundaries of genetic research, leading to advances that have a profound impact on medicine, agriculture, and biotechnology. Understanding this significance is not solely academic; it shapes experimental design and innovative solutions across multiple disciplines. In this section, we will explore three key applications: cloning vector construction, recombinant protein production, and gene function analysis.
Cloning Vector Construction
At the heart of molecular genetics, cloning allows scientists to generate copies of a specific DNA segment. Competent cells serve as the vessels for this technique. The basic principle involves inserting a piece of DNA into a cloning vector, which is typically a plasmid. Once this foreign DNA is introduced into competent cells, they take it up, resulting in numerous copies of that DNA segment being produced.
This process not only provides a means to study specific genes but also enables researchers to engineer these cloned segments for further experimentation. For instance, the construction of vectors that are tailored for particular applications, such as plasmids designed for expressing a protein in yeast or bacteria, expands our toolkit for genetic manipulation.
Recombinant Protein Production
Beyond merely cloning, competent cell transformation is pivotal in the realm of recombinant protein production. Proteins, often considered the workhorses of the cell, are synthesized based on the blueprints provided by DNA. By inserting the gene that encodes a desired protein into competent cells, we can utilize these biological factories to churn out large quantities of proteins that are otherwise hard to isolate from natural sources.
This method is particularly significant in the pharmaceutical industry, where proteins produced can be therapeutics or diagnostic agents. For example, insulin production was revolutionized with this technology, allowing for synthetic insulin to be produced efficiently. However, the selection of the right competent cells and appropriate expression systems is critical to maximizing yield and functionality of the produced proteins.
Gene Function Analysis
Understanding the role of specific genes in biological processes hinges on the ability to manipulate them effectively. Competent cell transformation allows for gene function analysis through various genetic techniques, such as gene knockout or knock-in studies. By inserting genetic material that can silence or replace a gene of interest, researchers can explore its function within the cellular context.
The exploration of gene functions might have far-reaching implications, including the identification of potential therapeutic targets for diseases or understanding metabolic pathways. Complicated interactions within biological systems can often be unraveled by systematically studying how altering a single gene impacts overall cellular behavior.
The critical role of competent cells in research extends beyond mere technical procedures; it is integral to advancing our understanding of biology itself.
Challenges in Competent Cell Transformation
The journey of introducing foreign DNA into cells using competent cell transformation is riddled with challenges. These obstacles not only impact the efficiency of the transformation process but also influence the outcomes of molecular biology experiments. Understanding these challenges is essential for researchers and practitioners aiming to optimize their transformation protocols and ensure reproducible results.
With the increasing complexity of biological systems and the demand for precision in genetic modifications, addressing the challenges in competent cell transformation has become more critical than ever. Enhancing the effectiveness of transformation techniques can lead to breakthroughs in cloning, gene therapy, and other significant biotechnological applications.
Low Transformation Rates
One of the most pressing challenges faced in competent cell transformation is the issue of low transformation rates. Low efficiency can stem from a variety of factors, including the choice of competent cell type, the method utilized for the transformation, and the quality of the plasmid DNA. For instance, if the competent cells are not freshly prepared or if they haven't been optimized for specific plasmids, the transformation rates can plummet.
To understand why low transformation rates are a concern, consider the implications in practical applications:
- Reduced Yield: When transformation rates are low, the yield of colonies carrying the desired plasmid is likewise diminished. This can lead to unnecessary time and resource expenditure in subsequent cloning steps.
- Inconsistent Results: Research relies heavily on reproducibility. Low transformation efficiency can introduce variability in experiments, leading to ambiguous results.
- Resource Inefficiency: For labs operating on tight budgets, especially in educational settings, every transformation that fails equates to wasted reagents and effort.
Strategies to combat low transformation rates include optimizing cell growth conditions, refining DNA preparation methods, and employing high-efficiency competent cells like those from the D-alpha or TOP10 strains which, while common, are known to yield better results under the right conditions.
Contamination Issues
Another significant challenge in the transformation process is contamination. Contaminants can come from various sources, such as reagents used, working environments, or even the competent cells themselves. This issue can manifest in different ways: decreased transformation efficiency, experimental failure, and even misleading results in downstream applications.
Contamination can be subtle, often going unnoticed at first. There are several key factors to consider:
- Source of Contaminants: Common contaminants include nucleases that degrade plasmid DNA, bacteria that compete with the transformed cells, and cross-contamination from other samples in the lab.
- Impact on Experiments: Contaminated cultures can lead to uneven growth, skewing results and interpreting experimental data. The presence of unwanted organisms can also complicate the purification process of recombinant proteins.
- Prevention Strategies: To minimize the risk of contamination, it’s important to maintain strict aseptic techniques. Regular cleaning of work surfaces, the use of sterile reagents, and proper storage of competent cells can significantly mitigate these risks.
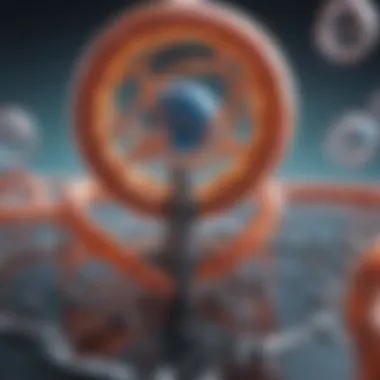
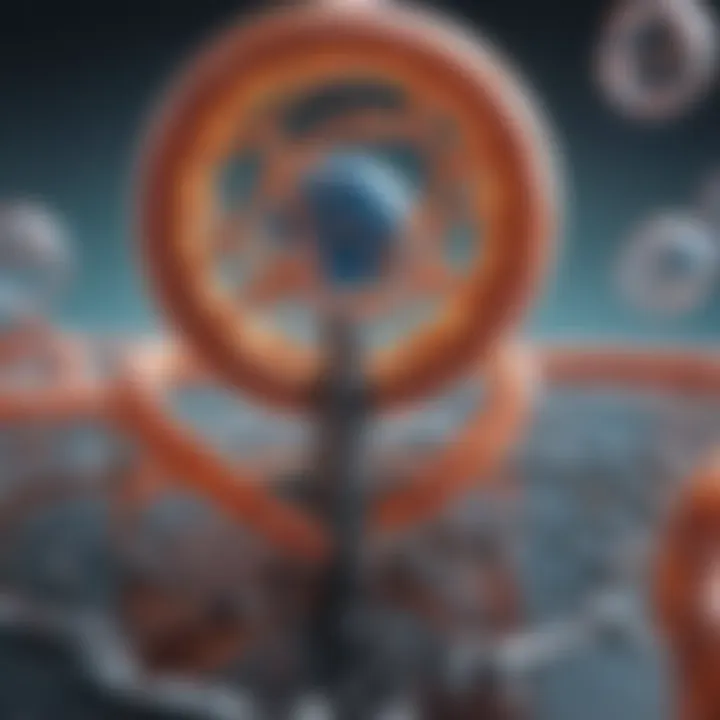
Maintaining a contamination-free environment is paramount to ensuring the integrity of molecular biology experiments.
In summary, both low transformation rates and contamination issues are substantial obstacles in competent cell transformation. By closely examining these challenges, researchers can adopt informed measures that enhance transformation efficiency, leading to more fruitful scientific inquiries and advancements in biotechnology.
Novel Techniques in the Field
In recent years, molecular biology has witnessed a significant shift, thanks largely to innovative techniques that have found their way into the realm of competent cell transformation. These novel methodologies are not just academic pursuits but are reshaping the very fabric of genetic engineering, pushing the boundaries of what’s achievable in research and application. Two standout approaches that have garnered attention are CRISPR gene editing and advanced electroporation systems.
CRISPR and Gene Editing
CRISPR (Clustered Regularly Interspaced Short Palindromic Repeats) technology stands at the forefront of genetic manipulation. Its advent has revolutionized how scientists approach gene editing and modifications. The beauty of CRISPR lies in its simplicity and efficiency, providing researchers with a powerful tool to make precise changes in the genome of organisms, including bacteria, plants, and animals.
From the perspective of competent cells, CRISPR facilitates several unique advantages:
- Targeted Modifications: Unlike traditional methods that often result in random integrations, CRISPR allows for accurate targeting of specific genes. This specificity reduces the likelihood of off-target effects, making experiments more reliable.
- Versatility: CRISPR systems can be adapted to target virtually any DNA sequence, enabling a wide range of applications in functional genomics, disease modeling, and even therapeutic developments.
- Efficiency: The CRISPR-Cas9 system has shown to vastly increase the transformation efficiency compared to classical techniques. This means that scientists can achieve desired outcomes with significantly lower amounts of DNA and in shorter timeframes.
However, it’s not all sunshine and rainbows. The utilization of CRISPR also brings considerations that researchers must navigate:
- Ethical Concerns: The power to edit genes also raises serious ethical questions, particularly when it comes to higher organisms or potential applications in humans. The debate surrounding “designer babies” looms large.
- Delivery Mechanisms: While CRISPR technology is robust, the successful delivery of the systems into competent cells can be tricky. Efficient transfection methods, like electroporation, remain essential to ensuring the process fully succeeds.
Advanced Electroporation Systems
Electroporation has evolved from a simple technique into a sophisticated method that incorporates cutting-edge technology. This method utilizes electrical pulses to open pores in the cell membrane, allowing for the entry of DNA into competent cells. Recent advancements have significantly enhanced the efficiency and reliability of this technique.
Here are some key aspects to consider with advanced electroporation systems:
- Customizable Protocols: Modern systems offer highly customizable settings tailored to specific cell types and experimental needs. Parameters like field strength, pulse duration, and pulse shape can be fine-tuned, making electroporation applicable across various biological systems.
- Scalability: Whether working with small lab-scale models or scaling up for industrial applications, advanced electroporation systems can accommodate varying batch sizes. This flexibility is particularly beneficial for large-scale recombinant protein production.
- Reduced Cell Death: Newer technologies have minimized cell death post-electroporation, a major challenge in earlier systems. Lower mortality rates mean that more competent cells survive the transformation processes, improving overall yields.
However, researchers are urged to keep in mind:
- Optimization Required: Not all cells respond uniformly to electroporation. Each cell line may require specific parameters for optimal results, often involving a series of experiments to determine the best conditions.
- Cost and Accessibility: Advanced electroporation systems might come with a hefty price tag, posing a barrier for smaller labs or institutions. This can limit the widespread adoption of these techniques, despite their potential.
Future Directions in Competent Cell Research
As the field of molecular biology continues to expand, the evolution of competent cells takes center stage. Investigating new frontiers for improving cellular transformation not only enhances existing methodologies but also opens doors to previously unattainable outcomes. Looking ahead, researchers and biotechnologists are exploring ways to push the boundaries of competent cell application, optimizing the critical processes involved in genetic manipulation.
Improving Transformation Protocols
Fine-tuning transformation protocols will likely bring substantial benefits to the science community. One way to achieve this is through the incorporation of high-throughput screening methods. These methods can rigorously assess various conditions affecting transformation rates, leading to more efficient protocols. Furthermore, using advanced computational models to predict optimal conditions based on factors like temperature and ion concentrations can streamline the entire transformation process.
Moreover, a focus on minimizing transformation's dependence on chemicals can create more environmentally friendly approaches. The exploration of natural alternatives or biodegradable substances might pave the way for safer and sustainable practices that do not compromise the efficiency of cell transformation.
It’s also worth examining the potential of integrating machine learning. By analyzing vast datasets, researchers can better understand underlying patterns and thus refine their protocols in a more systematic manner.
Synthetic Biology Approaches
The fusion of synthetic biology with competent cell research could alter the landscape of genetic engineering fundamentally. New synthetic techniques enable more precise manipulation of genetic sequences, allowing for the design of cells tailored for specific applications. For instance, scientists are increasingly looking toward creating custom plasmids that not only enhance stability but also facilitate higher transformation efficiency.
Additionally, using engineered competent cells can improve the production of recombinant proteins, significantly impacting industries like pharmaceuticals and agriculture. By creating cells with enhanced stress resistance, researchers can optimize yield while minimizing the loss during downstream processing.
The rise of CRISPR and other gene editing tools has also influenced synthetic biology approaches. These tools provide powerful abilities to modify genetic material precisely, resulting in improved cell functions. With more sophisticated synthetic biology techniques emerging, the potential to develop competent cells equipped with tailored functionalities is becoming more attainable.
"The future of competent cells is not about filling existing roles; it's about redefining what those roles can be."
In summary, the future directions in competent cell research hold thrilling promise. The integration of advanced methodologies and synthetic biology can drive this vital area of study forward. As such, it’s essential for educators, researchers, and industry professionals to remain informed about these developments, ensuring that they leverage these advanced techniques to improve their work in molecular biology. By embracing collaboration across disciplines, the molecular biology community can better navigate the challenges and seize the opportunities that lie ahead.
End
As we have explored throughout this article, several factors come into play during the transformation process. Each step, whether it be preparation methods or the introduction of plasmid DNA, is crucial. The efficiency of transformation can greatly influence cloning outcomes, protein expression levels, and ultimately the success of genetic experiments. For instance, knowing how to optimize your competent cell protocols can lead to higher yields and less waste of valuable resources.
A key benefit is the transformative power these cells hold in the face of challenges like low efficiency or contamination issues. Gaining insights into their applications enables scientists to tailor their approaches based on the specific needs of their research. For example, utilizing advanced techniques such as CRISPR or synthetic biology can push the limits of what is possible, facilitating groundbreaking discoveries.
Additionally, this article has taken a clear view of the future directions in competent cell research. By improving transformation protocols and exploring synthetic biology approaches, the scientific community can look forward to innovative solutions that will further enhance the capabilities of competent cells.
In summary, the significance of competent cell transformation goes beyond technique; it stands at the intersection of creativity and precision that drives progress in molecular biology. By mastering these concepts, researchers can unlock new potentials in genetic manipulation, ultimately contributing to the greater good of science and society.
Summary of Key Points
- Definition and Importance: Competent cells are essential for the introduction of foreign DNA and play a pivotal role in biotechnology.
- Methods: Various techniques—chemical and electroporation—highlight different ways to create competent cells.
- Factors Influencing Efficiency: The growth phase of cells, DNA quality, and environmental aspects are critical for successful transformation.
- Applications: Competent cells enable essential processes like cloning, protein production, and gene analysis.
- Challenges: Transformation rates and contamination issues pose ongoing challenges that require innovative solutions.
- Future Directions: Advancements in technology promise to improve protocols and expand the utility of competent cells in synthetic biology and beyond.
Final Thoughts on Transformation
The journey through competent cell transformation opens a multitude of avenues for exploration and innovation. Not only does it shed light on existing methodologies, but it also establishes a framework for future research initiatives. As we continue to delve deeper into the mechanics of competence, it is evident that the manipulation of DNA and the understanding of cell behavior will only grow in complexity.
In navigating this intricate landscape, one must appreciate the delicate balance between experimentation and theoretical understanding. The field is ripe for breakthroughs, and as advancements unfold, the implications of these transformational techniques are likely to reverberate across various domains of molecular biology. Staying informed and adaptable will be key for researchers as they forge ahead into the next era of genetic inquiry.