Understanding Linear Acceleration Radiation Explained
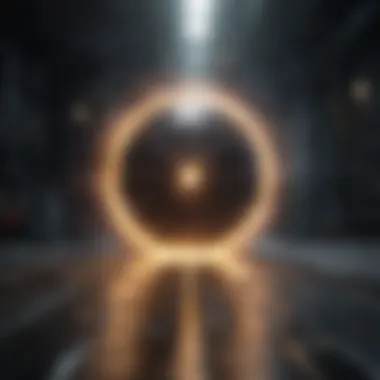
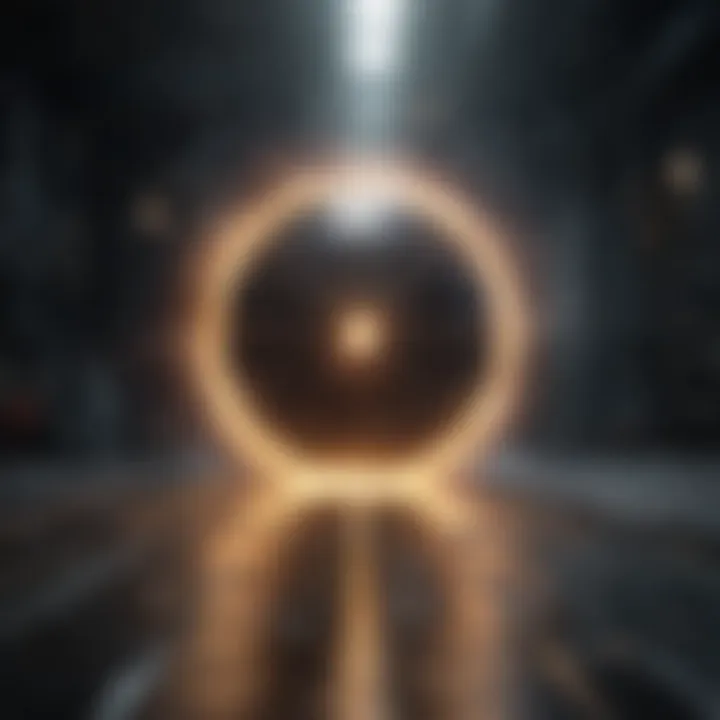
Intro
The phenomenon of linear acceleration radiation is rooted in the dynamics of charged particles. When these particles accelerate linearly, they emit electromagnetic radiation. This article explores the foundational principles underlying this complex interaction, its diverse applications, and its implications within the realm of contemporary physics.
The significance of understanding linear acceleration radiation arises from its relevance in various scientific domains. For example, its principles find applications in particle physics, astrophysics, and even within technology sectors that involve particle accelerators. This comprehensive exploration will unpack the key concepts, delve into the pertinent methodologies, and critically evaluate the existing research to elucidate this intricate subject.
Research Overview
Summary of Key Findings
Linear acceleration radiation, also known as Larmor radiation, indicates how a charge emits energy while being accelerated. The emitted radiation has critical importance in fields such as photon detection, astronomy, and advanced imaging technologies. Recent studies have further revealed that the energy characteristics of the emitted radiation are influenced by the rate of acceleration, the charge's mass, and its velocity. Findings suggest that as the acceleration increases, the intensity and frequency of the radiation emitted can vary significantly, revealing nuanced insights into particle behavior.
Methodologies Employed
Research about linear acceleration radiation employs a range of methodologies. Some of these include:
- Theoretical Models: Mathematical frameworks to simulate and predict behavior under linear acceleration, such as the use of Maxwell's equations.
- Experimental Approaches: Using particle accelerators like CERN's Large Hadron Collider to observe real-time interactions and radiation emissions.
- Observational Techniques: Utilizing telescopes and detectors that analyze radiation emitted from astronomical bodies, further informing our understanding of its impact.
In-Depth Analysis
Detailed Examination of Results
A thorough examination of the results from various studies shows that linear acceleration radiation varies significantly depending on the environment in which the charged particles exist. In high-energy settings, such as those in astrophysical environments, the radiation characteristics differ vastly from those in controlled lab settings. This understanding is crucial for theorizing about cosmic phenomena.
Comparison with Previous Studies
This exploration contrasts with earlier studies that primarily focused on static conditions of charge emission. Unlike previous models that emphasized static scenarios, recent findings illuminate dynamic aspects of particle behavior under acceleration. This shift highlights the need for further experimental validation to ensure that theoretical models align with observed data in practical applications.
"Understanding the intricacies of linear acceleration radiation opens new avenues for research and technological advancements, particularly in fields involving high-energy physics and astrophysics."
Prelims to Linear Acceleration Radiation
Linear acceleration radiation is fundamental to our understanding of both classical and modern physics. This radiation occurs when charged particles accelerate in a straight line. Understanding the mechanisms at play not only unveils the relationship between motion and radiation but also reveals the intricate dynamics that govern particle behavior. This section will discuss the definition and historical significance of this concept.
Definition and Overview
Linear acceleration radiation refers to electromagnetic radiation generated when charged particles, like electrons, accelerate in straight paths. This effect is critical in many areas of physics, as it underlies the principles of how charged elements emit energy in the form of radiation. It forms the basis of various technologies, including particle accelerators and medical imaging devices.
The radiation can be seen as a result of the changes in the electric and magnetic fields surrounding the moving particles. When these fields shift due to acceleration, they propagate through space, manifesting as electromagnetic waves. This phenomenon is not just a curiosity but a pivotal element in a plethora of scientific applications. It offers insights into the structure of matter and the behavior of particles at high energies.
Historical Background
The study of linear acceleration radiation has a rich history rooted in classical electromagnetism. Early theoretical foundations were laid by scientists such as James Clerk Maxwell, whose equations describe how electric and magnetic fields interact. The recognition that accelerating charges produce radiation emerged in the late 19th and early 20th centuries.
Significant advancements were made when researchers began to explore the applications of this phenomenon. For instance, in the 1940s, synchrotron radiation was identified when particles were accelerated in circular motions at high speeds, affirming that linear acceleration also had deep implications. These discoveries paved the way for the development of modern technologies, like synchrotron light sources used in experimental physics.
"Understanding linear acceleration radiation allows physicists to manipulate and utilize fundamental forces in innovative ways."
As a result of these historical advancements, the comprehension of how electricity and magnetism coalesce during particle acceleration unfolds new realms of inquiry. It has led to remarkable applications across various scientific fields, revealing the profound interconnections between energy, matter, and the forces of nature.
Fundamental Principles of Radiation
Understanding the fundamental principles of radiation is essential in the study of linear acceleration radiation. It lays the groundwork for comprehending how charged particles interact with their environment and the resultant effects of these interactions. This topic is not just academic; it carries significant implications in various scientific fields. For researchers and professionals alike, grasping these principles can lead to advancements in technology and improved methodologies in experiments.
One key aspect of radiation principles involves the study of electromagnetic radiation. This radiation arises from the motion of charged particles. Such particles emit energy when they are accelerated. This emission can be categorized based on frequency, wavelength, and energy, which are essential for applications in numerous domains, including medical imaging and astrophysics.
Moreover, the understanding of electromagnetic radiation serves as a bridge to analyzing the specific behaviors of charged particles under various conditions. By establishing the foundational knowledge outlined in this section, readers can appreciate how such principles inform experimental designs and technological innovations.
Nature of Electromagnetic Radiation
Electromagnetic radiation encompasses a spectrum of waves, from radio waves to gamma rays. This entire spectrum is characterized by its wavelength and frequency. When charged particles, such as electrons, are subjected to acceleration, they emit radiation as a byproduct of their change in motion.
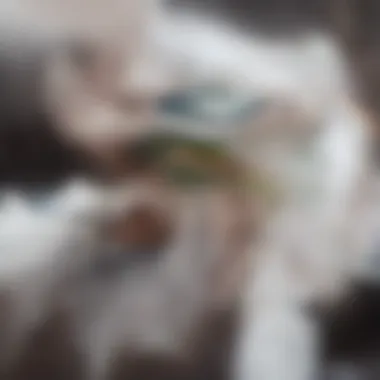
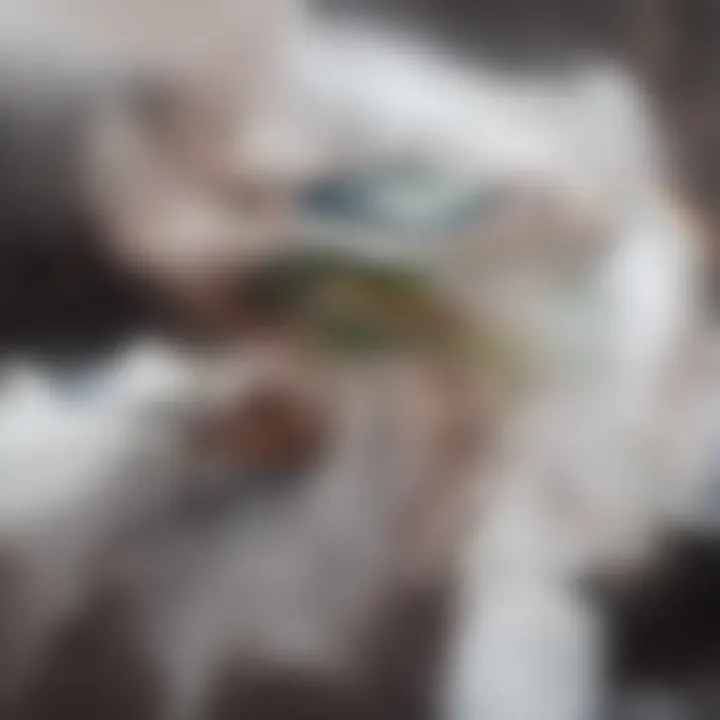
This emission has several critical characteristics that define its behavior:
- Wavelength: The distance between consecutive wave crests. This determines the type of radiation, such as visible light, radio waves, or X-rays.
- Frequency: The number of wave crests passing a point in one second. Higher frequencies correlate with higher energy and shorter wavelengths.
- Amplitude: The height of the wave, reflecting the intensity of the radiation emitted.
Understanding these attributes aids scientists in predicting how radiation behaves in different environments. The data gathered can be essential for applications ranging from radiation therapy in medicine to analyzing cosmic events in astrophysics. Insights into electromagnetic radiation guide further research into material interactions and technological applications.
Charged Particles and Their Behavior
Charged particles such as protons and electrons behave according to principles laid out by classical physics. Their behavior is heavily influenced by external forces, such as electric and magnetic fields. When these particles accelerate, they do not merely gain kinetic energy; they also emit radiation in the form of electromagnetic waves.
Key factors affecting charged particle dynamics include:
- Acceleration: The rate of change of velocity, leading to the release of energy in the form of radiation.
- Electric and Magnetic Fields: These fields interact with charged particles, modifying their trajectory and energy states, which can lead to different radiation outcomes.
- Energy Levels: As particles interact with fields and material, they can change energy levels, emitting or absorbing radiation accordingly.
The understanding of charged particles and their behavior under various influences is vital for advancements in several sectors. In particle physics research, this knowledge assists in particle detection and analysis, while in technology it underpins the development of devices such as accelerators.
"The fundamental behavior of charged particles not only reveals the intricate dynamics of radiation but also fosters innovation across scientific disciplines."
This information establishes a crucial foundation that supports further exploration in later sections of this article.
Mathematical Representation
Mathematical representation plays a crucial role in understanding linear acceleration radiation. It provides the tools to describe and quantify the phenomena associated with charged particles in motion. These mathematical frameworks enable researchers to predict how radiation is emitted, which is vital for applications in many fields, including medical imaging and particle physics.
Through equations and formulas, one can grasp complex interactions and behaviors that occur in different scenarios. Mathematical representation also aids in the validation of theoretical models. Therefore, mastering these concepts is essential for anyone interested in this field of study.
Key Equations and Concepts
A few key equations form the backbone of the mathematical understanding of linear acceleration radiation. One of the central equations governing this phenomenon is the Larmor formula. It expresses how the power radiated by a non-relativistic charged particle is related to its acceleration:
Where:
- ( P ) is the radiated power,
- ( q ) is the charge of the particle,
- ( a ) is the acceleration,
- ( c ) is the speed of light.
This equation shows the dependency of radiation on both charge and acceleration. The more a particle accelerates, the more radiation it emits. It simplifies the analysis of radiation in experimental setups and enhances the ability to anticipate outcomes.
Another important component is the electromagnetic field equations, outlined in Maxwell's equations. They describe how electric and magnetic fields propagate and interact with charges. Understanding these equations is fundamental to grasping how radiation spreads from a source and influences surrounding environments.
Calculating Radiation Emission
Calculating radiation emission requires a clear understanding of the processes involved. The key steps generally include:
- Determine Charge and Acceleration: Identify the properties of the charged particle involved.
- Apply the Larmor Formula: Use the equation mentioned earlier to calculate the power emitted due to acceleration.
- Consider Relativistic Effects: For particles moving at speeds close to the speed of light, one must adjust the calculations using the relativistic versions of Maxwell's equations and Larmor's formula, as they influence observed radiation.
- Data Analysis: Finally, empirical data from experiments must be collected and analyzed to ensure predictions align with real-world observations.
This methodical approach simplifies the process of understanding how radiation is emitted and provides researchers with insights necessary for different applications, ranging from technology to astrophysics.
Experimental Approaches
In examining linear acceleration radiation, the experimental approaches play a vital role in advancing our understanding of this intricate phenomenon. These methodologies not only allow researchers to test theoretical predictions but also to observe the various behaviors and characteristics of radiation emitted from charged particles. Understanding these approaches gives insight into their effectiveness, challenges, and potential to innovate further in both physics and applied technologies.
Setups for Studying Radiation
The setups used for studying radiation are of foremost importance. Designs of these experimental rigs can vary significantly depending on the specific type of radiation particles under investigation. Among the setups, particle accelerators like the Large Hadron Collider and synchrotrons are prominent.
Various detectors such as scintillation counters, ionization chambers, and semiconductor detectors are also critical components. These instruments need precision calibration for accuracy. Each setup aims to capture the details of the radiation emitted during the acceleration of charged particles to determine properties like energy, wavelength, and intensity.
Some key aspects to consider in the setup include:
- Location: The surrounding environment can greatly influence results, thus secluded areas are typically used to minimize interference from external radiation.
- Calibration: Regular calibration of instruments is necessary to ensure that data gathered are valid and reliable.
- Safety protocols: Operations must adhere to strict safety measures so as to safeguard both researchers and equipment from potentially hazardous radiation exposure.
Furthermore, flexibility in experimental setups often facilitates adaptability to new findings or theories in radiation physics.
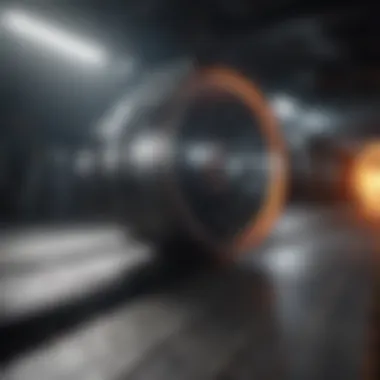
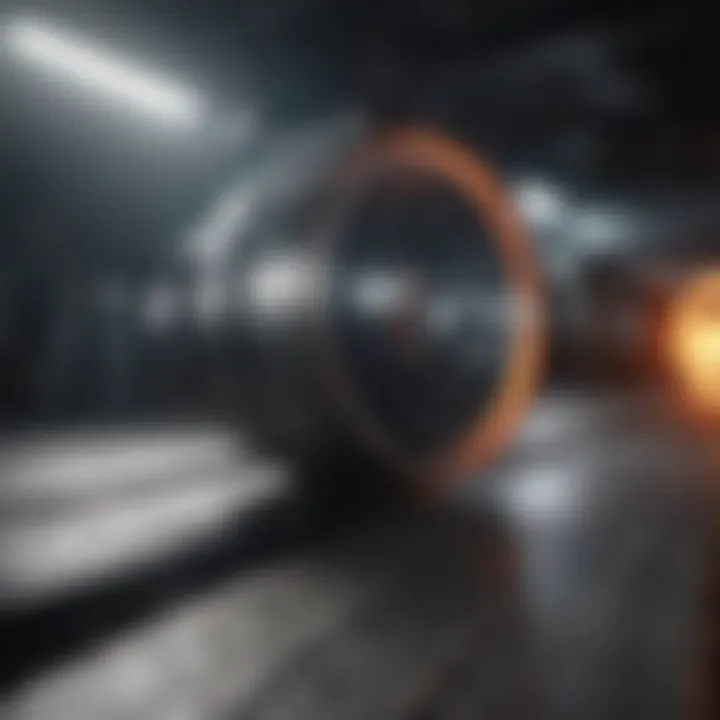
Data Collection and Analysis
Once the experimental setups are in place, the focus shifts to data collection and analysis. This phase is where raw data transforms into comprehensible information that can provide insights into the behavior of linear acceleration radiation.
Data collection involves systematic recording using automation or manual methods. Accuracy is essential in this phase, as any error can lead to flawed conclusions. Common data points include:
- Radiation intensity
- Particle energy levels
- Emission spectrum characteristics
After the collection phase, data analysis becomes critical. This often involves:
- Statistical Techniques: Tools applied to ensure that results are statistically significant. Techniques like regression analysis and hypothesis testing help draw meaningful conclusions from the data.
- Simulations: Computational models gauge the theoretical aspects against experimental findings, enhancing the understanding of observed results.
Additionally, data visualization tools help present findings effectively, aiding peer review and further research discussions.
Effective data collection and analysis are central to the integrity of experimental research in linear acceleration radiation.
Altogether, both setups for studying radiation and the subsequent data collection and analysis processes form a comprehensive infrastructure essential for significant advancements in linear acceleration radiation studies. The ongoing refinements in techniques and technologies will only bolster the capability to unveil new knowledge in this fascinating domain.
Applications of Linear Acceleration Radiation
Linear acceleration radiation has substantial significance across various fields, particularly in medicine, physics research, and astrophysics. Understanding these applications provides insight into how linear acceleration radiation contributes to advancements in technology and enhances scientific exploration.
In Medical Imaging
Medical imaging relies heavily on radiographic techniques that utilize linear acceleration radiation for diagnostic purposes. Techniques like X-rays and computed tomography (CT) scans employ the principles of radiation emitted from accelerated electrons. The primary strength lies in its capacity to produce detailed images of internal structures within the human body.
- Precision and Clarity: The ability to almost instantaneously capture detailed body images helps clinicians identify diseases and monitor health conditions effectively.
- Dosage Considerations: Medical professionals must manage radiation exposure to minimize risks while optimizing imaging quality. The advancements in technology allow for lower doses of radiation during imaging procedures, which improve patient safety.
- Emerging Techniques: Innovations such as digital radiography enhance the quality of images, further pushing the boundaries of diagnostic capabilities in modern medicine.
In Particle Physics Research
Particle physics has made considerable strides due to the study of linear acceleration radiation. In particle accelerators, charged particles, when accelerated to high velocities, emit radiation that can be analyzed to unlock the mysteries of fundamental forces and particles.
- Colliders: Facilities like the Large Hadron Collider (LHC) employ linear acceleration radiation as a key component in experiments. By observing the particles resulting from collisions, scientists can explore the properties of matter at subatomic levels.
- Detection Methods: Tools such as calorimeters and tracking detectors utilize radiation patterns to create a better understanding of particle interactions. Researchers leverage this data to refine models of particle physics.
- Theoretical Discoveries: The insights gained from experiments lead to the development of new theoretical frameworks and models, which can predict behaviors of particles under extreme conditions.
In Astrophysical Observations
Astrophysics benefits immensely from linear acceleration radiation to observe cosmic phenomena. The radiation emitted by charged particles can provide valuable insights into the processes occurring within stars, galaxies, and other astronomical structures.
- Cosmic Rays: Researchers study cosmic rays, high-energy particles that may originate from supernovae or active galactic nuclei. The radiation emitted as these particles travel to Earth offers clues about energetic processes in the universe.
- Radiation Telescopes: Instruments designed to capture the radiation emitted from linear acceleration in various celestial particles aid in understanding the composition and behavior of cosmic materials.
- Mapping the Universe: Data derived from observations assists in mapping cosmic events and the behavior of dark matterβan ongoing endeavor in understanding the universe's structure.
"The intersection of linear acceleration radiation with fields such as medicine, particle physics, and astrophysics illustrates the breadth of applications and the ongoing potential for scientific advancement."
The applications of linear acceleration radiation are diverse and profound. Whether in the realm of health diagnostics, particle exploration, or cosmic research, this branch of physics continues to reveal new insights and innovations.
Impact on Technology
The influence of linear acceleration radiation is profound within various technological domains. Understanding this radiation contributes significantly to advancements in both medical treatments and safety protocols. The impact encompasses several elements worth discussing.
First, in the realm of medical therapy, linear acceleration radiation plays a critical role in targeted treatments for cancer. Specifically, this kind of radiation is used in radiation therapy to deliver concentrated doses to tumors while minimizing damage to surrounding healthy tissues. By employing linear accelerators, healthcare professionals enhance treatment outcomes and improve patient quality of life. The precision of dose delivery mainly arises from an intricate understanding of how charged particles emit radiation when accelerated.
"The precision with which linear acceleration radiation can target tumors exemplifies the remarkable intersection of physics and medicine."
Second, linear acceleration radiation has significant implications for radiation safety and standards. Facilities that utilize this technology must adhere to strict regulations to ensure the safety of both patients and personnel. This includes proper shielding, monitoring of radiation exposure levels, and regular maintenance of equipment to prevent accidental over-exposure. The continual assessment and advancement of safety protocols are necessary to uphold these standards, while simultaneously enabling the exploration of innovative applications in various fields.
In addition, understanding linear acceleration can lead to better innovations in technology that further benefits society. For instance, sectors such as astrophysics and particle physics rely on the foundational concepts associated with radiation produced from accelerated charged particles. This understanding paves the way for future technologies that may enhance imaging, detection systems, and even new materials developed for different applications.
Theoretical Implications
Understanding the theoretical implications of linear acceleration radiation is crucial for advancing knowledge in both classical and quantum physics. This section delves into the nuanced connections between established theories and emerging ideas, drawing attention to how they influence current research and applications.
Relation to Classical Electrodynamics
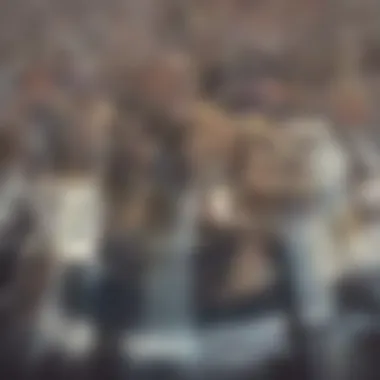
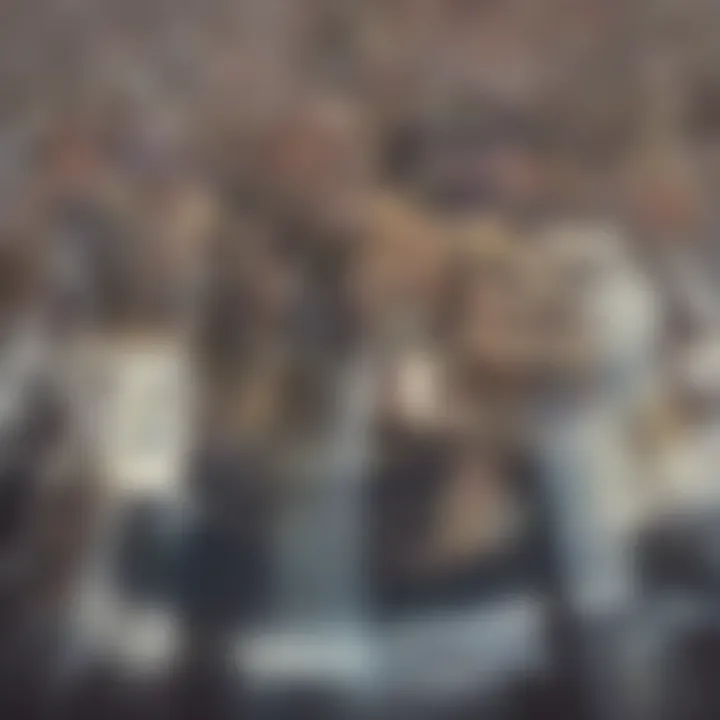
Classical electrodynamics serves as the foundation for understanding how charged particles emit radiation when they undergo linear acceleration. According to the principles outlined by James Clerk Maxwell, electric charges in motion create electric and magnetic fields, which propagate as electromagnetic waves. The implications of this are manifold:
- Accelerating Charges: When a charged particle changes its velocity, there is a resultant emission of radiation. This scenario is particularly relevant in particle accelerators, where particles like electrons or protons are repeatedly accelerated to high energies.
- Larmor Formula: This formula quantifies the power radiated by non-relativistic charges in motion and provides a clear connection between acceleration and radiation output. Understanding this relationship is vital for engineers designing devices that harness or mitigate radiation from charged particles.
- Field Interactions: Classical electrodynamics not only explains the mechanics of radiation but also its interaction with surrounding fields. This knowledge is crucial when developing technologies like MRI or in radiation therapy, where field interactions can significantly impact treatment efficacy.
Quantum Considerations
In contrast to classical views, quantum mechanics introduces complexities into the understanding of linear acceleration radiation. Charged particles are not merely point charges; they also exhibit wave-particle duality, making their behavior under acceleration far more intricate:
- Photon Emission: In quantum terms, accelerated electrons emit photons due to transitions between energy states. This phenomenon underlines many applications, including lasers and synchrotron radiation facilities.
- Quantum Electrodynamics (QED): This theoretical framework extends classical principles, accounting for interactions between light and matter at a quantum level. It provides profound insights into how radiation can be quantized and exploited in advanced technologies.
- Threshold Energy Levels: Quantum mechanics raises considerations related to energy levels and their significance in radiation emission. In contexts like electron-positron annihilation, understanding these thresholds is essential for predicting radiation types and intensities.
"The intersection of classical electrodynamics and quantum mechanics offers profound insights into the mechanisms driving linear acceleration radiation."
Challenges in Research and Application
The study of linear acceleration radiation presents significant challenges for researchers and practitioners alike. Understanding these challenges is crucial for advancing the field and ensuring the safe and effective application of the principles involved. The complexities of measurement, technology, and the interplay of theoretical concepts complicate the path of inquiry. This section delves into specific measurement challenges and technological limitations that shape current research and application.
Measurement Challenges
Accurate measurement of radiation from accelerated charged particles is a primary challenge within this domain. One of the primary reasons for these difficulties is the need for highly sensitive instrumentation. The radiation emitted can often be weak, particularly in scenarios involving low-energy particles, making detection arduous. Moreover, various external factors can affect measurements.
- Environmental Interference: Electromagnetic noise from surrounding equipment or even natural sources can skew results. This interference necessitates careful calibration and shielding of instruments.
- Distance Dependency: The intensity of radiation diminishes with distance. Measuring at appropriate proximity is often necessary, yet it poses risks to researchers and equipment.
- Time Variability: Radiation emission can change rapidly, requiring real-time monitoring solutions to capture accurate data. Systems must be robust enough to handle this variability to produce reliable analyses.
Inadequate measurement techniques can lead to erroneous conclusions, thus stifling advancements in technology and understanding of linear acceleration radiation.
Technological Limitations
The technology employed for studying linear acceleration radiation is not without its constraints. Various instruments and equipment form the backbone of radiation research. However, limitations in these tools can hinder progress significantly.
- Resolution of Detecting Instruments: Many traditional detectors may not provide the resolution needed for detailed studies. More advanced technologies, such as high-resolution spectrometers, often come with prohibitive costs and complexities in operation.
- Data Processing Capacity: The vast amounts of data generated during experiments necessitate sophisticated data management and analysis systems. Current computational limitations might slow down research, making it imperative for researchers to integrate more advanced computing approaches.
- Integration of Emerging Technologies: New technologies like artificial intelligence show promise for improving analysis. However, their integration is still in nascent stages and requires further development before they can be relied upon.
The future of research in linear acceleration radiation hinges on overcoming both measurement and technological hurdles. Addressing these challenges will pave the way for significant advancements in understanding and applications, making this pursuit critical.
Future Directions in Linear Acceleration Radiation Study
The study of linear acceleration radiation is an evolving field with significant potential for advancement. Researchers are constantly exploring new areas and techniques that could enhance our understanding of the nature of radiation emitted from charged particles. Identifying future directions is crucial for pushing the boundaries of what is currently known. This section emphasizes the prospective domains of research, their implications for technology, and the overall benefits of continued exploration in this area.
Emerging Research Areas
In recent years, various emerging research areas have gained attention in the context of linear acceleration radiation. Some of these include:
- Nanotechnology Applications: The integration of nanotechnology with radiation studies opens doors for miniaturized, high-precision applications in medical imaging and treatment methods. Researchers are investigating how nanoscale materials can manipulate radiation to improve outcomes.
- Plasma Physics: Understanding the interactions between charged particles in plasma can unlock new profiles of radiation emission. This can have implications for fusion energy research and astrophysical phenomena.
- Advanced Detection Methods: The development of more sensitive and accurate detection methods is vital. Research is being conducted on new sensors and imaging techniques that can measure radiation with greater precision, leading to better data collection and analysis.
- Interdisciplinary Approaches: Collaboration between fields such as chemistry, biology, and materials science with radiation physics offers insights that can lead to innovative applications, particularly in environmental monitoring and safety.
Potential Innovations in Applications
The potential innovations driven by the research in linear acceleration radiation are vast. Some notable areas include:
- Improvements in Radiation Therapy: As the understanding of linear acceleration radiation deepens, innovations in targeted radiation therapies for cancer treatment can be developed. Tailoring radiation doses more precisely can minimize damage to surrounding healthy tissues while maximizing tumor destruction.
- Enhanced Imaging Techniques: With advancements in radiation detection and analysis, medical imaging modalities like X-rays and CT scans might become sharper and more informative, aiding in early diagnosis and treatment planning.
- Space Exploration Technologies: As missions to outer space increase, understanding radiation sources will be crucial for protecting astronauts from harmful rays. Innovations could arise in the form of better shielding materials or radiation monitoring systems.
- Environmental Applications: Innovations might also extend to environmental sciences. Understanding radiation emissions can help monitor pollution levels and track hazardous materials, allowing for improved environmental health strategies.
Continued advancements in linear acceleration radiation research not only enrich the scientific community but also contribute to practical solutions in medicine, technology, and environmental safety.
In summary, the future of linear acceleration radiation research looks promising. Emerging areas of study and potential innovations in applications provide numerous opportunities to enhance existing technologies and develop new approaches. This sustained interest and investment in research will ultimately lead to significant contributions to both science and society.
The End
The conclusion serves a vital role in summarizing the extensive discussion presented throughout this article. It encapsulates the key themes surrounding linear acceleration radiation, emphasizing its significance in advancing our understanding of high-energy physics and the behavior of charged particles. The relevance of this topic cannot be overstated, as insights gained from this realm have far-reaching implications across numerous fields including medical imaging, particle physics, and technology development.
Summary of Key Points
In reviewing the crucial elements discussed, several points stand out which are integral to the theme of this article:
- Understanding the Basics: A foundational grasp of linear acceleration radiation is crucial for appreciating the more complex concepts that build on this knowledge.
- Applications in Diverse Fields: The practical applications highlighted include advancements in medical technologies such as radiology and new methodologies in particle physics research.
- Research Challenges: Recognizing the obstacles faced in experimental setups and data analysis ensures a realistic perspective on ongoing research.
- Future Directions: Emerging areas in this field, including advancements in quantum technology and their implications, showcase the continued evolution and relevance of linear acceleration radiation.
Final Thoughts on the Future of Research
Looking ahead, the trajectory of research in linear acceleration radiation appears promising. As technology advances, the capabilities to explore this field will only improve. New frameworks in quantum mechanics could reveal deeper insights into the behavior of emitted radiation and its interaction with matter.
Moreover, interdisciplinary collaborations are expected to yield innovative applications of linear acceleration radiation in both practical technologies and theoretical exploration. Therefore, engaging with this field from different perspectives will likely enhance our understanding and promote breakthroughs that can benefit society at large.
In summary, the ongoing exploration of linear acceleration radiation stands at a critical juncture in modern science, meriting attention and further inquiry.