Understanding Lithium-Ion Car Batteries: Key Insights

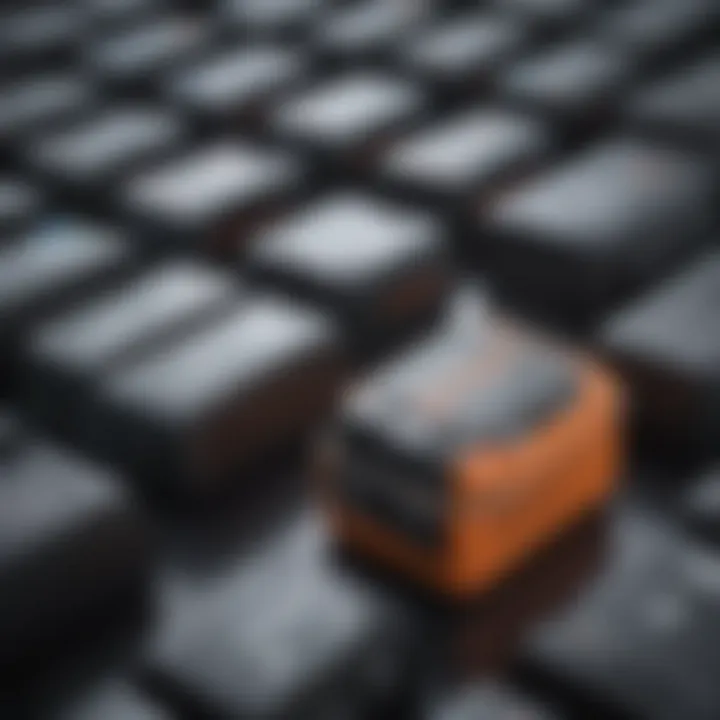
Intro
In a world increasingly leaning toward electrification, understanding the core components and functioning of lithium-ion car batteries becomes paramount. Not only do these batteries fuel the movement toward sustainable energy, but they also represent a pivotal element in the evolution of automotive technology. By laying a foundation of knowledge about their inner workings, one can better appreciate both the advantages and challenges these power packs present in modern electric vehicles (EVs).
The significance of lithium-ion batteries is not just confined to their functionality. As we dive deeper, we will touch on their composition, including crucial materials like anodes, cathodes, and electrolytes. Understanding these elements provides clarity on how these batteries convert chemical energy into electrical energy, ultimately powering the vehicles we drive daily.
The narrative will also elucidate matters like thermal management strategies to prevent overheating and ensure safety standards are met. Additionally, as we move further into a landscape of technological advances, we shall consider the strides being made in recycling these batteries, thereby reducing their environmental footprint. Engaging with this content is essential for a comprehensive grasp of the future of transportation and energy reliance.
Through this exploration, the article aims to foster heightened awareness and appreciation of the intricacies involved in lithium-ion technology, crucial not only for students and researchers but also for enthusiasts eager to understand more about the automotive industry's shift towards sustainable solutions.
Foreword to Lithium-Ion Batteries
Lithium-ion batteries are pivotal to modern technology, especially within the automotive realm. These batteries, known for their efficiency and compact design, power everything from cellphones to electric cars, making them a fundamental piece of contemporary life. In an age where sustainability is paramount, understanding the intricacies of lithium-ion batteries becomes essential for students, researchers, and industry professionals alike. This section is a gateway to delve into the historical background and the growth trajectory of these batteries, setting the stage for comprehending their composition and functionality.
Historical Context
The genesis of lithium-ion technology dates back to the late 20th century, when the quest for innovative electrical energy storage solutions began to intensify. Researchers were grappling with the limitations of nickel-cadmium batteries, which were heavy, toxic, and suffered from memory effects. The landscape shifted significantly in 1980 when John Goodenough, Rachid Yersin, and their teams discovered that lithium cobalt oxide could serve as an efficient cathode material. This discovery paved the way for the first commercial lithium-ion batteries, which hit the market in the early 1990s.
"The rise of lithium-ion bulk storage technology marked a turning point in portable electronics, enabling new possibilities for design and function."
As smartphones and laptops became ubiquitous, the demand for lightweight, rechargeable batteries skyrocketed. Lithium-ion technology stepped up, meeting consumersโ appetite for better performance. Although early iterations faced challenges, improvements over the years have led to enhanced safety and longevity, which is crucial in todayโs fiercely competitive market.
The Rise of Electric Vehicles
Fast forward to the 21st century, we find ourselves amidst an electric vehicle revolution. With climate change concerns looming large, the automotive industry has embraced lithium-ion batteries as a cleaner alternative to fossil fuels. The hybrid and fully electric cars utilize these batteries not only for propulsion but also for energy recovery during braking, thus enhancing efficiency.
Several factors have spurred the rise of electric vehicles:
- Environmental awareness among consumers
- Technological advancements reducing battery costs
- Government incentives promoting clean energy transportation
This electrification of transport has catalyzed significant research and investment in lithium-ion technology, pushing boundaries of battery size, capacity, and safety. As we strive to chart a sustainable future, understanding the fundamentals of lithium-ion batteries becomes not just academic, but a necessity in revolutionizing mobility and energy applications.
Basic Science of Lithium-Ion Batteries
Understanding the basic science behind lithium-ion batteries is crucial as it lays the groundwork for comprehending their composition and functionality. This knowledge not only helps in grasping how electric vehicles operate, but also sheds light on the efficiency, safety, and environmental considerations linked to battery technology.
The core principle behind lithium-ion batteries is embedded in electrochemistry, where chemical energy converts into electrical energy through redox reactions. This foundational concept supports the entire framework of battery operation, impacting both everyday user experience and broader technological innovations.
Electrochemistry Fundamentals
Electrochemistry is the heartbeat of lithium-ion battery technology. Essentially, it revolves around the movement of lithium ions between the anode and cathode during discharge and charge cycles. In simpler terms, think of it as a relay race where lithium ions sprint back and forth, completing a loop that powers your device.
During discharge, ions travel from the anode, passing through the electrolyte and through the separator, landing at the cathode. When charging, the process reverses. This flow of ions is what generates electricity, enabling electrical appliances and electric vehicles to function efficiently. This movement is facilitated by the electrolyte, which allows ions to travel while acting as a barrier for electrons, ensuring that they follow the correct path without creating shorts.
Components of a Lithium-Ion Battery
The construction of a lithium-ion battery consists of several key components, each playing a vital role: anodes, cathodes, electrolytes, and separators. Understanding these elements in detail leads to a richer comprehension of how these batteries work, what makes them effective, and even their limitations.
Anodes
Anodes are one of the two electrodes in a lithium-ion battery and are typically made of graphite, a material chosen for its ability to intercalate lithium ions. One major characteristic of graphite-based anodes is their high capacity to store lithium ions during charging. This trait makes them a prevalent choice in electric vehicles.
However, while graphite anodes can deliver substantial energy density, they often struggle with rapid charging cycles, which can cause degradation over time. This characteristic of performance versus longevity is a crucial aspect to consider when examining a battery's efficiency and service life.
Cathodes
Cathodes are the counterpart to anodes in the battery direction of charge flow. They are often constructed of lithium metal oxides, such as cobalt oxides, nickel manganese cobalt (NMC), or lithium iron phosphate (LiFePO4). A significant feature of cathodes is their capacity to attain higher voltage levels, resulting in increased energy density. This makes them highly advantageous for applications requiring longer range and performance.
Nonetheless, the trade-off includes a challenging manufacturing process and higher costs. Particularly, cobalt-based cathodes raise concerns about supply chain ethics and environmental impact, bringing important considerations into discussions about sustainable battery technology.
Electrolytes
Electrolytes serve as the medium for lithium ion transport between the anode and cathode. Generally classified into liquid or solid forms, electrolytes massively influence the overall performance of the battery. Liquid electrolytes, like lithium salt dissolved in organic solvents, tend to offer excellent ionic conductivity, which is critical for performance.
On the other hand, solid electrolytes are emerging as a promising alternative, boasting enhanced safety by eliminating flammability risks associated with liquids. Nevertheless, they may present challenges regarding ionic conductivity and manufacturability, highlighting an ongoing area of research and development in lithium-ion technology.
Separators
Separators are thin membranes that keep the anode and cathode apart while allowing ions to flow freely. Their main role is to prevent short circuits while maintaining high ionic conductivity. Typically composed of porous polyethylene or polypropylene, separators are crucial in ensuring battery safety and performance.
Their unique feature lies in their ability to act as a barrier, maintaining the integrity of the cell structure. However, whichever path they seem to take, they also require careful design to ensure they donโt become a bottleneck in ion flow, as this can decrease battery efficiency.
Incorporating these components effectively ensures that lithium-ion batteries can meet the demands of modern applications, driving the need for continuous research into improving material properties and structural designs. By understanding these basics, one can appreciate the remarkable technological feats that lithium-ion batteries enable in today's world.
Anode Materials
The role of anodes in lithium-ion batteries is pivotal, dictating both the performance and longevity of the battery itself. Anode materials essentially determine how energy is stored and released during the charging and discharging cycles. In electric vehicles, where battery efficiency is paramount, understanding anode materials becomes essential. The choice of anode impacts not only the energy density but also the charge/discharge rates, cycle stability, and safety of the battery.
Types of Anodes Used
Graphite-Based Anodes
Graphite-based anodes are like the bread and butter of lithium-ion technology. They've been the go-to choice for a long while, primarily due to their affordability and good performance characteristics. The structure of graphite allows for lithium ions to intercalate, which is a fancy way of saying that lithium can insert itself between graphite layers, enabling efficient charge storage.
One key characteristic of graphite-based anodes is their high specific capacity, usually around 372 mAh/g. This means they can store a significant amount of lithium, allowing for extended battery life and range in electric vehicles. Furthermore, they offer a relatively good cycle stability, meaning they can be charged and discharged multiple times without significant degradation.
However, it isn't all sunshine and rainbows. The major drawback lies in their performance at high charge rates. When you push them too hard, say, during rapid charging, the process can become less efficient and may lead to overheating. This can be a cause for concern in high-performance electric vehicles, where fast charging is often marketed as a strong selling point.
Lithium Titanate Anodes
On the other end of the spectrum are lithium titanate anodes, which present a different set of advantages. While they might not pack as much energy density as graphite, their key characteristic is ultra-fast charging capability. Lithium titanate anodes can handle rapid cyclingโlike charging from zero to full in mere minutesโwithout showing signs of degradation.
This high-power capability stems from their unique crystal structure, which allows lithium to move in and out quickly. Such performance makes them a favoured option for applications requiring quick energy bursts, like in certain forms of public transport or emergency response vehicles. However, they're not without their downsides. The major disadvantage of lithium titanate is their lower energy density, typically around 175 mAh/g. This means that, in terms of pure storage, they donโt hold a candle to graphite-based anodes.
Performance Characteristics
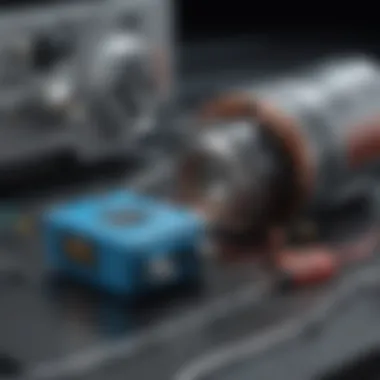
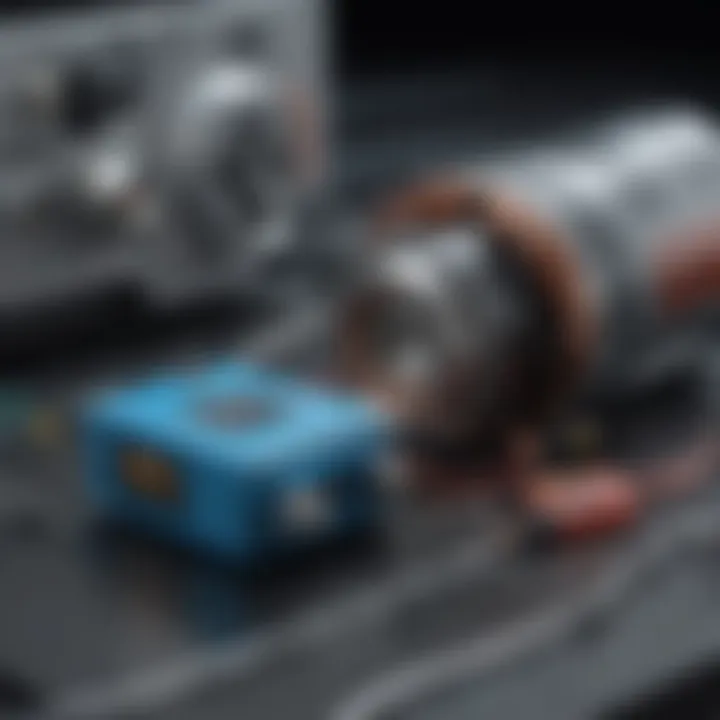
When analyzing performance characteristics, several factors come into play. Both anode types play a critical role in defining the batteryโs efficiency, longevity, and even safety factors. Different applications may benefit from different types of anodes, leading to trade-offs that designers and engineers have to make carefully. In summary:
- Graphite-Based Anodes:
- Lithium Titanate Anodes:
- High specific capacity
- Good cycle stability
- Challenges with rapid charging and heat management
- Exceptional charge/discharge rates
- Long cycle life
- Lower energy density compared to graphite
In the end, the choice between these materials hinges on the specific requirements of the vehicleโs design and use-case scenarios. As we look towards advancements in battery technologies, understanding the nuances of these anode materials will continue to be pivotal for researchers, industry players, and consumers alike.
Cathode Materials
When dissecting the intricate world of lithium-ion car batteries, cathode materials hold a pivotal role. These materials are not just filler; they are the backbone that supports the performance and efficiency of a battery. Essentially, the cathode is where lithium ions exit the electrolyte and enter the anode during discharging, shaping how energy is stored and utilized. This section zeroes in on common cathode materials and their distinct characteristics, bringing to light the pros and cons associated with each type.
Common Cathode Materials
Cobalt Oxides
Cobalt oxides, particularly lithium cobalt oxide (LiCoO2), are among the most established cathode materials in consumer electronics and electric vehicles. The primary characteristic of cobalt oxides is their high energy density, which essentially means they can store a significant amount of energy in a relatively small footprint. This makes them a favored choice for applications where space and weight are at a premium.
However, while cobalt oxides are lauded for their efficiency, they do come with drawbacks. One major aspect is the ethical concern surrounding cobalt mining, often linked to dangerous labor conditions and environmental impact. Furthermore, cobalt oxides can also lead to stability issues at elevated temperatures, potentially compromising safety. Hence, while they are beneficial for their energy density, the ethical and safety considerations surrounding cobalt have stirred discussions in both the industry and academia.
Nickel Manganese Cobalt (NMC)
Nickel Manganese Cobalt (NMC) is a hybrid material that has started to gain traction in various battery applications, including electric vehicles. A hallmark of NMC is its balanced composition, providing a fusion of high energy density from nickel, stability from manganese, and improved conductivity from cobalt. This mix produces a performance profile that appeals to manufacturers looking for a well-rounded option.
NMC's unique feature is its scalability โ manufacturers can tweak the ratio of nickel, manganese, and cobalt to tailor specific performance requirements. However, one drawback is that increasing the nickel content raises manufacturing costs and can lead to thermal management challenges. This trade-off between performance and cost is important for stakeholders touching both the engineering and financial sides of battery production.
LiFePO4
LiFePO4, or Lithium Iron Phosphate, has carved out a niche in the market, particularly regarding safety and longevity. Its key characteristic is thermal stability, meaning it is less prone to overheating, which is a significant advantage when considering battery safety. Furthermore, LiFePO4 batteries boast a long cycle life, making them ideal for applications where longevity is critical, such as in large-scale energy storage systems and electric buses.
The unique feature of LiFePO4 is its relatively low cost compared to cobalt-based materials. Despite this advantage, it does paint a different picture regarding energy density. LiFePO4 usually delivers less energy per unit mass than its counterparts, meaning more space is required to achieve the same energy capacity. Thus, while it provides safety and cost benefits, it's not the go-to option for scenarios needing maximized energy density.
Impact on Battery Performance
The choice of cathode material directly affects the performance of lithium-ion batteries in numerous ways. From energy capacity to thermal stability and lifespan, each materialโs properties play a key role in determining how a battery operates in the real world.
- Energy density: This dictates how much energy can be stored, influencing the range of electric vehicles.
- Thermal stability: Affects safety, as materials like LiFePO4 present a lower risk of thermal runaway.
- Cycle life: Refers to how many charge-discharge cycles a battery can undergo before performance diminishes.
In summary, selecting the right cathode material is not just a technical decision; it's a multifaceted consideration that balances performance, safety, cost, and ethical implications. The material ultimately chosen can shape the future of electric vehicle technology, emphasizing the need for ongoing research and development in this field.
Electrolytes in Lithium-Ion Batteries
Electrolytes play a crucial role in the operation of lithium-ion batteries. They are the medium through which lithium ions move between the anode and cathode, which is fundamental for the batteryโs charging and discharging processes. Without electrolytes, the flow of these ions would be obstructed, resulting in a failure to generate electrical energy. Consequently, understanding electrolytes is indispensable for grasping how these batteries function and what tools are needed to enhance their performance and reliability.
Types of Electrolytes
Liquid Electrolytes
Liquid electrolytes are the traditional choice in lithium-ion batteries. They are generally composed of lithium salt dissolved in a solvent, typically a mixture of organic solvents like ethylene carbonate and dimethyl carbonate. One of the standout features of liquid electrolytes is their excellent ionic conductivity, enabling lithium ions to move with relative ease. This characteristic results in high efficiency when charging and discharging the battery.
However, there are downsides too. Liquid electrolytes can be flammable, posing risks especially when a battery is damaged or improperly handled. This flammability is a serious concern and necessitates stringent safety measures during battery design and use. Additionally, due to constant exposure to heat and other factors, liquid electrolytes can degrade over time, leading to reduced performance.
Solid Electrolytes
Solid electrolytes, on the other hand, are emerging as a promising alternative to their liquid counterparts. These electrolytes consist of solid materials, like certain ceramics or polymers, that conduct ions. One key characteristic of solid electrolytes is their stability and safety. Unlike liquid electrolytes, they are non-flammable, significantly reducing the risk of fire or explosion.
The unique feature of solid electrolytes is that they can enable all-solid-state batteries, which often provide higher energy density. This means more energy can be packed into a smaller space. However, solid electrolytes face challenges in terms of lower ionic conductivity compared to liquid electrolytes and can be more expensive to produce, which has hindered widespread adoption in commercial applications.
Role of Electrolytes in Battery Function
The primary role of electrolytes in lithium-ion batteries is facilitating the transport of lithium ions, which directly impacts the battery's capacity, longevity, and efficiency. When the battery charges, lithium ions move from the cathode through the electrolyte to the anode, and when discharging, they flow back towards the cathode. This movement is critical for the reaction processes that generate electrical energy.
Moreover, the choice of electrolyte influences the operational temperature range of the battery. Variability in temperature can affect the battery's efficiency and lifespan, as some electrolytes perform better under certain thermal conditions. Therefore, selecting the right electrolyte is essential not just for functionality but also for optimizing performance and ensuring reliability over the battery's operational life.
The importance of electrolytes cannot be overstated; they are the lifeblood of lithium-ion batteries, ensuring that energy flows efficiently and safely between the anode and cathode.
In summary, electrolytes serve as the backbone of lithium-ion batteries, impacting everything from safety to performance. As the industry pushes toward more effective and sustainable solutions, ongoing research and development in the area of electrolytes will likely unveil promising advancements.
Battery Management Systems
Battery Management Systems (BMS) play a crucial role in ensuring the longevity, efficiency, and safety of lithium-ion batteries. While batteries are often seen as straightforward devices, the complexities of their operation make BMS indispensable. They function as the nervous system of the battery, monitoring and managing various parameters to optimize performance.
Purpose and Role of BMS
At its core, a Battery Management System is designed to monitor the health and status of a battery pack, ensuring that each cell within it operates safely and efficiently. This encompasses several key responsibilities:
- Voltage Regulation: Each cell within a battery has a specific voltage range. The BMS ensures that individual cell voltages remain within safe parameters, preventing overcharging or excessive discharging.
- State of Charge (SoC) Estimation: BMS determines how much energy is remaining in the battery, which aids in usage and charging decisions.
- Fault Detection: By continuously monitoring the batteryโs status, the system can identify irregularities like short circuits or overheating.
Such functions are fundamental for electric vehicles, where even a minor battery issue can lead to performance drops or safety hazards.
Key Features of Efficient BMS
An effective BMS is characterized by several essential features:
Cell Monitoring
Cell monitoring is a vital aspect of a BMS. It keeps tabs on each individual cell's voltage, temperature, and current. The real-time data gathered helps in assessing the overall health of the battery pack.
- Key Characteristic: The primary function of cell monitoring is to ensure that all cells are balanced in terms of charge. This balance is crucial for maintaining overall efficiency and prolonging battery life.
- Benefits: Cell monitoring enables timely detection of any cells that might be underperforming, allowing for corrective actions before they degrade the battery pack's overall health.
- Unique Feature: A robust monitoring system can integrate diagnostic capabilities that alert users even before issues escalate. This proactive approach can save costs associated with battery replacements and repairs.
Temperature Control
Temperature control is another critical feature of a BMS that helps manage the thermal environment of the battery. Lithium-ion batteries are sensitive to temperature variations, as extreme conditions can lead to performance inefficiency or worse, catastrophic failure.
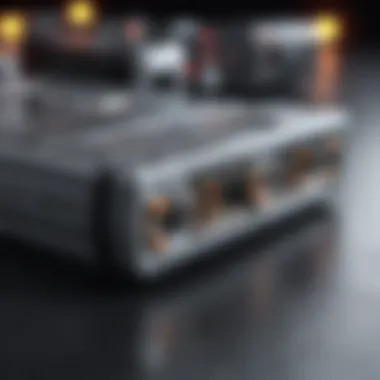
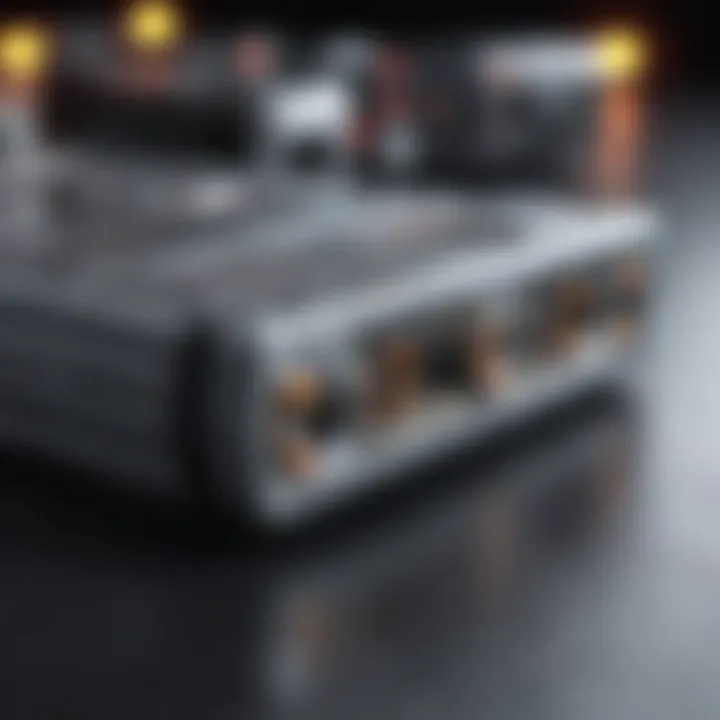
- Key Characteristic: Efficient temperature control systems typically employ sensors that detect temperature changes and respond by activating cooling or heating mechanisms as necessary.
- Benefits: The primary advantages of proper temperature management are prolonged battery life and enhanced safety. By maintaining optimal conditions, a BMS can minimize the risks associated with thermal runaway.
- Unique Feature: Some modern BMS incorporate advanced materials and technologies for more efficient heat dissipation, allowing for a more compact design while maintaining performance integrity.
In summary, BMS are not just supplementary components but essential systems that enhance battery performance, ensure safety, and extend the lifespan of lithium-ion batteries in electric vehicles and beyond.
Effective BMS can significantly differentiate battery technology in a competitive market, making them a focal point in the development of future battery innovations.
Charging Mechanisms
Charging mechanisms are at the heart of lithium-ion battery technology. The way energy flows into these batteries directly impacts both their lifespan and efficiency. A good charging system ensures that the battery performs well, allowing users to get the most out of their electric vehicles. Understanding charging methods helps in optimizing the performance of the battery, while also enabling better energy management.
Types of Charging Processes
Constant Current Charging
Constant Current Charging is one of the widely used methods when it comes to recharging lithium-ion batteries. In this system, the charging circuit outputs a steady current, which is particularly important during the initial phase of charging. This approach helps in quickly replenishing the battery's charge, ramping up the energy flow right from the start. This method stands out because it allows the battery to fill up quickly without undue stress on the cells.
A key characteristic of this method is that it operates at a fixed current, meaning that it fuels the battery until it reaches a predetermined voltage. The simple nature of Constant Current Charging makes it a popular choice across various applications, particularly in electric vehicles.
Advantages of Constant Current Charging include:
- Rapid Charging: Speeds up the process by getting the battery charged quickly.
- Currant Management: Can efficiently manage different battery sizes without tweaking charging parameters.
However, this method also has its drawbacks. Too much current can lead to excessive heat generation, which might pose a risk of thermal runawayโa critical concern in lithium-ion batteries. While the method is largely beneficial, attention must be given to cooling mechanisms during the charging process.
Constant Voltage Charging
On the other hand, Constant Voltage Charging takes a steadier approach. Once the battery reaches its target voltage during charging, the system maintains that voltage while adjusting the current according to the battery's state. This method transitions to a lower current to prevent overchargingโan essential feature when it comes to safeguarding battery integrity.
A defining trait of Constant Voltage Charging is that it focuses on the voltage regulation instead of maintaining a constant current, unlike the previous method. This balance significantly contributes to increasing the lifespan of the battery, as it prevents the potential overcharging scenarios seen in constant current methods.
Its unique features include:
- Voltage Preservation: Protects the battery from over-voltage conditions.
- Current Flexibility: Adapts based on the battery's state, providing a gentler charging experience.
Despite its advantages, this method can sometimes result in slower charging times compared to the constant current approach, which might be considered a disadvantage for users wanting quick turnaround times.
Factors Influencing Charging Efficiency
Charging efficiency of lithium-ion batteries is not solely dependent on the mechanisms mentioned. Several factors come into play, affecting how quickly and safely a battery can be charged.
- Temperature: Extreme heat or cold can slow down the charging process or risk damage to the battery.
- Battery Age: Older batteries may not hold charge as efficiently as new ones, influencing the overall charging effectiveness.
- Charger Quality: Well-designed chargers contribute significantly to optimizing the charging cycle.
In summary, both Constant Current and Constant Voltage charging processes have their respective pros and cons. Understanding the specifics of each method can assist in making informed choices, ultimately driving better results in the performance of lithium-ion batteries, especially in electric vehicles.
In a nutshell, effective charging mechanisms can make or break the performance of lithium-ion batteries, presenting opportunities for enhancementsโthis domain continues to evolve rapidly.
Thermal Management in Lithium-Ion Batteries
Thermal management plays a critical role in the functionality and lifespan of lithium-ion batteries. As these batteries charge and discharge, they generate heat. This increase in temperature can affect not only the performance of the battery but also its safety. Proper thermal regulation is essential to maintain optimal operating temperatures, prevent failures, and enhance efficiency. By controlling the heat generated, manufacturers can improve charge cycles and extend battery life.
Importance of Thermal Regulation
Thermal regulation is vital to maintaining the balance between performance and safety in lithium-ion batteries. Elevated temperatures can lead to several issues such as:
- Reduced Efficiency: High temperatures can cause the internal resistance of the battery to rise, leading to a loss in energy efficiency during charge and discharge cycles.
- Degradation of Materials: The extreme heat can adversely affect the chemical compounds used in both anodes and cathodes, leading to quicker deterioration.
- Safety Hazards: One of the most concerning risks is thermal runaway, where an increase in temperature can accelerate further reactions, leading to fires or explosions.
This makes the understanding and implementation of robust thermal management systems imperative.
Methods of Heat Management
Efficient heat management strategies have been developed, primarily falling under two categories: Passive Cooling and Active Cooling Systems. Each has its own characteristics and applicable scenarios.
Passive Cooling
Passive cooling utilizes natural methods to dissipate heat without requiring additional energy input. Its key characteristic is simplicity. With no need for mechanical components, passive systems rely on the battery design, incorporating materials that enhance thermal conductivity and airflow.
For example, using materials like aluminum can help in distributing heat more evenly across the battery pack.
- Advantages:
- Disadvantages:
- Cost-effective, requiring minimal investment in complex cooling systems.
- Lower maintenance, as there are no mechanical parts that can fail.
- Limited cooling capacity, which may not sufficiently handle extreme heat conditions.
- Less effective in densely packed battery configurations common in electric vehicles.
Active Cooling Systems
In contrast, active cooling systems employ mechanical methods to manage heat. This means they involve pumps, fans, or even chillers to actively remove heat from the battery cells. The main advantage here is that active systems can significantly lower temperatures more effectively.
- Key Feature:
- Advantages:
- Disadvantages:
- Control over the cooling process, allowing for adjustments based on real-time temperature monitoring.
- More efficient temperature regulation, helping maintain optimal charging conditions.
- Better suited for high-performance applications, especially in electric vehicles with high energy demands.
- Increased complexity, leading to higher costs in terms of both installation and maintenance.
- More components increase the potential for something to go wrong, thus requiring regular checks and balances.
Having a solid thermal management strategy ensures that lithium-ion batteries can perform at their best while remaining safe under various operating conditions.
Overall, thermal management is not just an accessory but a necessity. For electric vehicle manufacturers and battery designers, understanding and optimizing these systems will continue to shape the efficiency and reliability of lithium-ion batteries.
Safety Considerations
When we open the hood on the world of lithium-ion batteries, safety stands out like a neon sign. These powerhouses, while driving innovation in the electric vehicle sector, come with a set of safety considerations that must not be swept under the rug. Understanding these risks and implementing effective strategies to mitigate them is crucial for manufacturers and consumers alike.
The importance of this topic canโt be overstated. As electric vehicles become a staple on our roads, the potential hazard of lithium-ion batteries cannot be ignored. From manufacturing to end-of-life disposal, the mishaps can lead to catastrophic failures. It's not just about keeping cars on the road; itโs also about ensuring that their power sources donโt pose threats to human life or the environment.
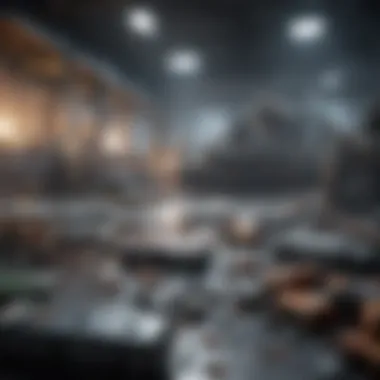
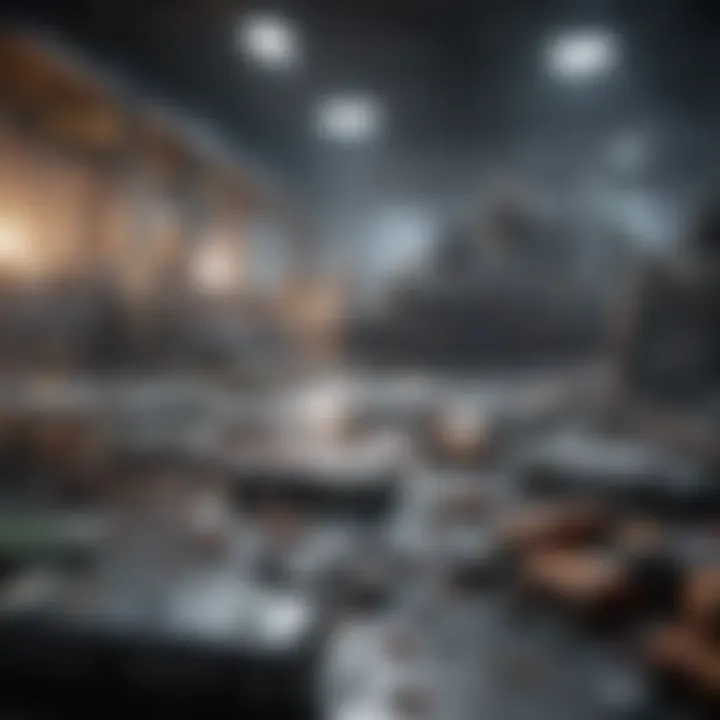
Common Risks with Lithium-Ion Batteries
Lithium-ion batteries, while efficient, are not without their flaws. Two prevalent risks come up quite often: thermal runaway and short circuits.
Thermal Runaway
Thermal runaway refers to a chain reaction that can occur when the battery cells overheat, leading to further heating and eventual failure, often resulting in fires or explosions. The key characteristic of this phenomenon is that itโs difficult to control once it begins, making it a point of concern for battery safety.
Why is thermal runaway a hot topic for our discussion? Because it starkly highlights the necessity for efficient thermal management systems in electric vehicles. As more lithium-ion batteries hit the streets, understanding the unique features of thermal runaway becomes critical.
It's essential to note that while the advanced design of modern lithium-ion batteries has significantly reduced the risk of thermal runaway, it is not entirely eliminated. Factors contributing to this risk include:
- Manufacturing defects
- Improper charging cycles
- Physical damage
Advantages? Well, understanding it means better designs and safety protocols can be developed to avert potential disasters.
Short Circuits
Short circuits represent another primary risk factor in lithium-ion battery safety. This occurs when the positive and negative terminals of a battery come into direct contact, allowing current to flow where it shouldnโt. The key characteristic of this risk is its unpredictability; a small puncture might lead to a massive failure in mere seconds.
Short circuits are significant because they can lead to overheating, fires, and even explosions. Itโs not just the battery itself thatโs at risk, but the electronic systems connected to it as well.
The unique feature of short circuits lies in their suddenness. Unlike thermal runaway, which builds over time, a short circuit can happen out of the blue due to:
- External factors like water intrusion
- Internal damage from manufacturing errors
In terms of advantages or benefits of understanding this risk, knowledge fosters better protective measures, safeguarding not just the batteries but entire vehicles. The more we know, the better we can manage these incidences.
Mitigation Strategies
To tackle these risks effectively, several mitigation strategies can be put in place:
- Robust BMS (Battery Management Systems): Ensures optimal performance and safety.
- Regular Maintenance: Helps catch any potential issues before they escalate.
- Education and Training: Essential for those who handle or work with these batteries.
"Understanding the risks associated with lithium-ion batteries is as crucial as the technology itself."
Environmental Impact and Sustainability
Understanding the environmental impact and sustainability of lithium-ion car batteries is essential, especially in today's context where climate change and energy resources are increasingly pressing concerns. As the automotive industry shifts toward electric vehicles, itโs vital to look at how these batteries are designed, used, and ultimately disposed of or recycled. This perspective addresses a myriad of crucial elements, benefits, and considerations that come with the growing reliance on lithium-ion technology.
Life Cycle Assessment of Lithium-Ion Batteries
Life cycle assessment (LCA) is a systematic approach for evaluating the environmental impacts associated with all stages of a product's life, from raw material extraction through production, use, and disposal. For lithium-ion batteries, conducting a thorough LCA is pivotal for identifying areas where improvements can be made.
- Raw Material Extraction: Lithium, cobalt, and nickel are key components of these batteries, and their mining practices have raised environmental concerns. Excessive water usage and toxic waste generation are common challenges in regions like Bolivia and the Democratic Republic of Congo, where these minerals are sourced.
- Manufacturing: The battery production process demands a significant amount of energy. Factories that produce lithium-ion batteries often run on fossil fuels, which means that the manufacturing phase can be carbon-intensive. Transitioning to renewable energy sources for this production could significantly reduce the carbon footprint.
- Usage: When it comes to usage, lithium-ion batteries are known for their efficiency and longevity. Their ability to recharge multiple times minimizes waste and contributes positively to energy conservation efforts. This efficiency has a direct impact on the overall environmental footprint of electric vehicles.
- End-of-Life Phase: The end-of-life stage involves disposal and recycling, which are critical for sustainability. Batteries that are not properly disposed of can leach harmful chemicals into the environment. Therefore, establishing protocols for how to manage used batteries is paramount for mitigating ecological harms.
A comprehensive LCA, therefore, offers insights into areas for innovation and sustainability enhancements in battery technology.
Recycling Processes
Recycling lithium-ion batteries is a vital component in addressing the environmental challenges they pose. It not only alleviates the pressure on raw material extraction but also minimizes overall waste. The recycling processes can be dissected into several key stages:
- Collection and Transportation: Before recycling begins, old batteries must be collected. This step often involves collaboration with local authorities or organized programs to ensure batteries are gathered and transported safely.
- Sorting: Upon reaching recycling facilities, batteries are sorted based on their chemistry and structure. This helps determine the most effective recycling method for each type.
- Deconstruction: In this phase, batteries are dismantled to separate the useful materials, such as lithium, cobalt, and nickel, from the plastic and metal casings. Techniques like pyrometallurgical or hydrometallurgical processing are employed depending on the material being extracted.
- Refinement: After deconstruction, the raw materials undergo refinement to remove impurities. This step ensures that the materials can be reused in the battery production cycle.
- Closed-loop Cycle: Ideally, extracted materials can be reintroduced into new battery production, creating a closed-loop system that conserves resources.
Recycling not only mitigates the environmental impact of lithium-ion batteries but also plays an essential role in making electric vehicles environmentally sustainable in the long run. However, efficiency and technological advancements in recycling processes continue to evolve, aiming for higher recovery rates and reduced operational impacts.
"The journey of a battery doesnโt end once it powers a vehicle. How we handle it after service determines the true sustainability of the whole lifecycle."
Future of Lithium-Ion Technology
The progression of lithium-ion battery technology is crucial as it closely aligns with our need for sustainable energy solutions, especially in the automotive sector. As electric vehicle production ramps up, innovations in battery technology become more than just a competitive edge; they represent the future of transportation itself. Understanding emerging trends in this domain is not simply about staying currentโit's about anticipating the needs of a rapidly evolving market. These enhancements can lead not only to better battery performance but also to a reduced environmental footprint, addressing both consumer and manufacturer concerns alike.
Emerging Materials
Sodium-Ion Batteries
Sodium-ion batteries have been gaining traction as an alternative to traditional lithium-ion options. One significant aspect of these batteries lies in their use of sodium, which is much more abundant and less costly compared to lithium. This affordability offers a intriguing proposition for large-scale applications, helping to address the economic constraints tied to lithium supplies.
A notable characteristic of sodium-ion technology is its potential for greater safety and thermal stability. Unlike lithium, sodium is less reactive, which might reduce risks associated with overheating and thermal runawayโa common concern in lithium-ion batteries.
However, sodium-ion batteries aren't without their disadvantages. Current energy density levels lag behind their lithium counterparts; they simply do not store as much energy per weight. This limitation means they may not be a silver bullet for powering electric vehicles just yet, especially when high performance is non-negotiable.
Solid-State Batteries
Solid-state batteries represent a groundbreaking shift in the design and operation of battery technology. Instead of using a liquid electrolyte, these batteries employ a solid electrolyte, which can lead to substantial improvements in safety and efficiency. The fundamental aspect of solid-state technology is the increased energy density it offers. With the potential for lighter batteries that can hold more energy, this innovation could extend the driving range of electric vehicles, addressing one of the most significant concerns among consumers today.
An important unique feature of solid-state batteries is their ability to operate over a wider temperature range, which opens up new applications beyond just vehiclesโthink home energy storage or portable electronics.
Still, solid-state batteries come with their own set of challenges. Manufacturing processes are more complex, leading to higher production costs. This factor presents a barrier to entry into the mass market, despite the promise these batteries hold for the future.
Trends in Battery Development
As we examine the trends emerging within the battery sector, several themes become apparent:
- Integration of AI: Battery management systems are increasingly utilizing artificial intelligence to optimize charging cycles and prolong life.
- Recycling Innovations: Companies are working on technologies to improve the recycling process of old batteries, making it more efficient to recover valuable materials.
- Research in Alternative Materials: Beyond lithium and sodium, researchers are investigating other materials that could make batteries more sustainable and cost-effective.
"Battery technology is not just about chemistry; itโs about rethinking how we store energy for a sustainable future."
These developments mark not just technological advances but also a societal shift toward cleaner, more efficient ways of harnessing energy. With the future of battery technology playing a critical role in the automotive industry, its growth could result in wider implications for energy policy and environmental regulations across the globe.
Finale
Lithium-ion batteries have become the backbone of modern electric vehicles, driving not only the automotive industry but also the shift towards sustainable energy solutions. Understanding the composition and functionality of these batteries is crucial for multiple reasons. It not only informs manufacturers about optimal material choices but also equips consumers with the knowledge necessary to make informed decisions. Considering the complexities involved in battery design and performance, a well-rounded insight into their workings enables advancements in efficiency and safety.
The Path Forward
As we forge ahead, the future of lithium-ion technology is poised for significant transformations. Researchers are actively exploring alternatives to current materials, aiming to make batteries lighter and more efficient. For instance, a shift towards solid-state batteries could resolve some inherent shortcomings of liquid electrolytes, such as volatility and leakage. The quest for new materials like sodium-ion batteries also shows promise, potentially minimizing dependency on lithium and contributing to resource sustainability. Moreover, approaches such as recycling waste batteries effectively can lessen environmental impact. These advancements indicate that we can engineer solutions that are not just more powerful but also environmentally friendly.
Impact on the Future of Mobility
The ripple effects of innovations in lithium-ion batteries extend well beyond individual electric vehicles. As battery technology evolves, we can expect to see a marked enhancement in driving ranges, reduction in charging times, and overall affordability of electric vehicles. This transformation could lead to a surge in EV adoption, ultimately reshaping urban landscapes and contributing to a decline in greenhouse gas emissions. Infrastructure development, such as the establishment of widespread charging stations, can complement these changes, paving the way for a seamless transition to electric mobility. The future is bright, and as we embrace these technologies, we inch closer to a cleaner, more efficient world, positioning ourselves at the forefront of sustainable transport.