Exploring the Link Between DNA and Amino Acids
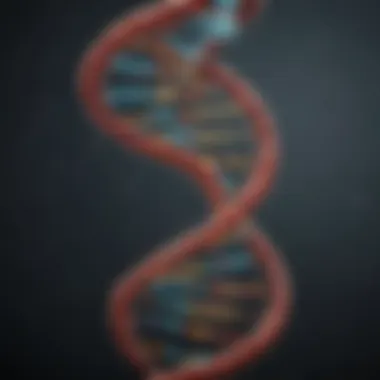
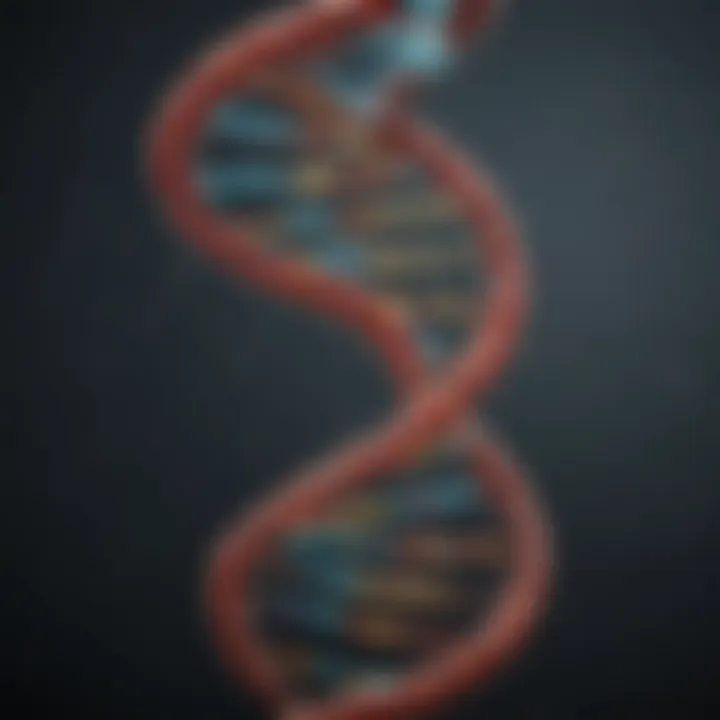
Intro
At the heart of biology lies an extraordinary dance between molecular structures that dictate life itself. Every living organism is shaped by DNA—the double helix that carries genetic instructions, synonymous with life's blueprint. But what happens when these instructions are translated into proteins, the workhorses of cells? Understanding this profound connection is essential, not only for unraveling the mysteries of life but for advancing fields like genetic engineering and medicine.
The journey from DNA to amino acids is intricate and meticulously orchestrated, involving a series of processes that ensure genetic information is accurately relayed into functional proteins. From the quiet whispers of the nucleus where DNA resides, to the bustling ribosomes where protein synthesis occurs, each step is a crucial element of life's machinery. This article aims to shed light on the key mechanisms driving this transformation, spotlighting the significance of transcription, translation, and the roles of various molecules involved.
In the ensuing sections, we will journey through the structural wonders of DNA, delve into the intricacies of RNA, and examine the implications of mutations. Specifically, we will explore how these elements culminate in the formation of polypeptides, ultimately shaping the cell's functions. Such insights not only enrich our understanding of molecular biology but also underscore the relevance of this knowledge in the burgeoning fields of biotechnology and medicine.
Research Overview
When we examine the processes at play between DNA and amino acids, it becomes clear how foundational these components are to all living systems. A comprehensive exploration of this relationship reveals key findings that have unfolded over decades of research.
Summary of Key Findings
- The Central Dogma: The flow of genetic information adheres to a general framework—DNA transcribes to RNA, which subsequently translates to proteins. This framework provides a roadmap for understanding genetic expression.
- Codons and Anticodons: Codons, three-nucleotide sequences on mRNA, serve as templates for amino acids. Corresponding anticodons on tRNA ensure that the correct amino acid is added to the growing polypeptide chain, highlighting the precision of this translational process.
- Mutational Impact: Mutations, even slight changes in the genetic code, can result in profound effects on protein function and, by extension, on organisms’ traits or health. Understanding these consequences has implications in disease pathology and evolutionary biology.
Methodologies Employed
A multitude of methodologies underpins the study of the DNA-to-amino acid journey. Techniques such as:
- Sequencing Technologies: With advancements like next-generation sequencing, researchers can map out genomes swiftly, providing insights into genetic variability and mutation rates.
- In Vitro Translational Systems: These controlled environments enable detailed investigation into the translational processes, helping in the understanding of initiation, elongation, and termination phases.
- Bioinformatics Tools: The use of specialized software helps in analyzing vast amounts of genomic data, giving rise to new hypotheses and approaches in molecular biology research.
"Understanding how DNA translates into proteins isn't just an academic exercise; it is foundational for precision medicine, agricultural advancements, and unraveling genetic diseases."
This multifaceted approach to research not only strengthens our grasp of molecular intricacies but also opens avenues for innovative applications in various scientific fields.
Understanding DNA Structure
Grasping the characteristics of DNA's structure is crucial in unraveling how it holds the blueprints for life. This structure is not merely a formality but rather the very essence of genetic information storage and transmission. In this article, we will explore its important characteristics, which come into play during the processes leading from DNA to amino acids, marking the foundation for concepts like protein synthesis.
The Double Helix Model
At the heart of DNA's architecture lies the iconic double helix model. Picture a twisted ladder: this visual captures the essence of DNA quite effectively. The two strands of DNA coil around each other, held together by complementary base pairing between nucleotides. Each rung of that twisted ladder comprises pairs of nitrogenous bases—adenine pairs with thymine, and cytosine with guanine.
This harmonious pairing plays a pivotal role during replication and transcription, ensuring that the genetic code remains intact. The beauty of the double helix lies not only in its shape but in its simplicity and efficiency in information storage. When cells divide, they can easily replicate their DNA, passing on accurate genetic information to the next generation.
"The double helix structure is not just an architectural feature of DNA; it embodies the principles of stability and fidelity in genetic transcription and replication."
Nucleotides: The Building Blocks
Understanding nucleotides is essential for grasping the full picture of DNA’s function in our cells. Nucleotides can be thought of as the fundamental units or building blocks that make up the DNA strands. Each nucleotide consists of three components: a phosphate group, a five-carbon sugar (deoxyribose), and a nitrogenous base. The sequence of these bases is what encodes genetic information.
To put it simply, think of nucleotides as letters in a long sentence that determine the meaning of the whole text. Variation in their order results in different genes and, consequently, different traits in an organism. This intricate coding system reflects the mechanisms by which genetic instructions are translated into functional products, like proteins.
Each time a cell divides or needs to create proteins, the nucleotides play their part in ensuring that accurate copies of the genetic code are made available. As you can see, without an understanding of these basic components, one can hardly appreciate the complex ballet that leads from DNA to the creation of amino acids—our cellular building blocks.
The Role of Genes in Protein Synthesis
Understanding the role of genes in protein synthesis is at the heart of molecular biology. Genes, located on the DNA, serve as the blueprint for constructing proteins, which play a pivotal role in virtually every biological process. Without genes, life as we know it would simply not exist. They dictate not only the structure but also the functions of proteins, which are essential for cell structure, function, and regulation of the body's tissues and organs.
Genetic Coding: From DNA to Proteins
The process of translating genetic information from DNA into proteins begins with the concept of genetic coding. This coding is ultimately expressed through codons, which are sequences of three nucleotides that correspond to specific amino acids. Each gene contains instructions that are transcribed into messenger RNA (mRNA) and subsequently translated into a chain of amino acids during protein synthesis.
In simpler terms, think of DNA as the final manuscript of a novel, where every word and punctuation mark matters to convey the intended story. The mRNA acts as a copy of that manuscript, summarizing it for easy digestion. Ribosomes, the cellular machines, then read this script and assemble the corresponding amino acids, creating a protein based on the genetic instructions.
Some key aspects of genetic coding include:
- Triplet Codons: Each combination of three nucleotides in mRNA translates to a specific amino acid.
- Start and Stop Codons: These are essential markers in the coding sequence that signal where the protein synthesis should begin and end.
This evolutionary mechanism ensures that the genetic information is accurately translated, maintaining the integrity of protein function across generations.
Hierarchical Structure of Genes
When discussing the structure of genes, it's important to visualize a well-organized library. Just as a library is structured by bookshelves, categories, and sections, genes are structured hierarchically. A gene can be viewed as an intricate file within this vast collection of information, but this file consists of various components, each serving a distinct purpose.
- Regulatory Elements: These are like librarians guiding which genes should be read at what times. They regulate the expression of genes, ensuring that proteins are produced when and where they are needed.
- Exons and Introns: Exons are the chapters of the file that contain the actual instructions while introns can be seen as the blank spaces in between. During the transcription process, introns are spliced out, allowing only the necessary information to be sent out as mRNA.
- Promoters and Enhancers: Think of these as bookmarks. Promoters are regions that enhance transcription initiation, while enhancers can facilitate a higher level of expression by binding factors that help in transcription despite being located far from the actual gene.
The hierarchical organization ensures that the genes function efficiently, adapting according to environmental conditions and specific cellular demands. Without this structure, protein synthesis would falter, affecting vital biological functions.
Genes are not just segments of DNA; they are sophisticated control systems that influence the cell’s machinery to adapt and respond to changes. Understanding their role in protein synthesis is crucial for comprehending how life operates at a molecular level.
Transcription: The First Step
Transcription serves as the launch pad for the complex journey from DNA to amino acids, acting as a crucial first step in the intricate tapestry of protein synthesis. This process transforms the coded information encoded in DNA into messenger RNA (mRNA), which ultimately guides the synthesis of proteins in the ribosomes.
Understanding transcription is essential because it not only dictates the protein-coding genes' expression but also sets the stage for the next pivotal phases of gene expression. The implications stretch across various fields, from molecular biology to biotechnology, making this understanding foundational for students, researchers, and professionals alike. At its core, transcription is more than a simple copying process; it’s a highly regulated mechanism that ensures the correct genes are expressed at the right time in the right cells.
Molecular Mechanics of Transcription
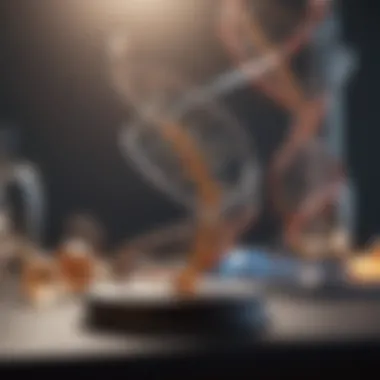
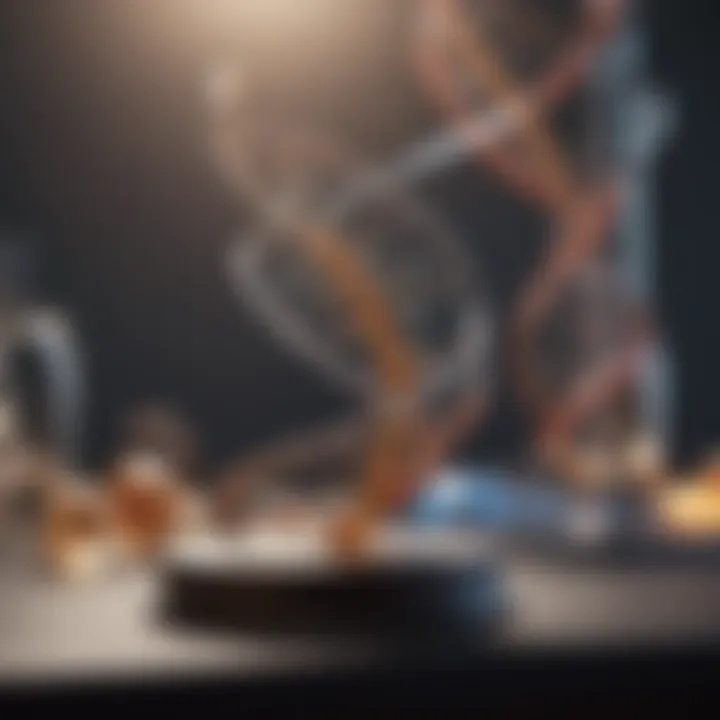
Delving into the nitty-gritty of transcription reveals a sophisticated ballet of molecular players. It begins when an enzyme called RNA polymerase binds to specific sequences known as promoters located at the beginning of a gene. This binding is critical; it’s like a conductor signaling the orchestra to get ready.
Once the RNA polymerase plugs in, it unwinds a small portion of the DNA double helix, exposing the bases of the gene. As RNA polymerase moves along the DNA strand, it synthesizes a single strand of RNA by catalyzing the addition of ribonucleotides, complementary to the DNA template. To give a clearer picture, here's how the process unfolds:
- Initiation: RNA polymerase binds to the promoter, forming a transcription complex.
- Elongation: As the enzyme progresses, it unwinds DNA, pairing complementary nucleotides and extending the growing mRNA strand.
- Termination: The enzyme reaches a termination signal, prompting it to detach from the DNA, leaving a newly synthesized mRNA strand.
This entire sequence is carefully regulated, with various factors influencing when and how much mRNA is produced. The nuances of this regulation ultimately decide how genes are expressed in different contexts.
The Role of RNA Polymerase
At the heart of transcription is RNA polymerase, a multifaceted enzyme that not only synthesizes RNA but also ensures accuracy and regulation during this critical phase of gene expression. Without RNA polymerase, the conversion of genetic information from DNA to mRNA would not be feasible.
There are several types of RNA polymerases in eukaryotic cells, each with its specific responsibilities. For instance, RNA polymerase II is primarily responsible for synthesizing mRNA, while RNA polymerase I and III deal with ribosomal RNA and transfer RNA, respectively.
This enzyme operates under a refined process, responding to various signals within the cell, ensuring that genes are expressed when needed. Factors such as transcription factors, which can turn genes on or off, interact with RNA polymerase to modulate gene expression. This dynamic interplay allows cells to adapt quickly to internal and external changes, playing a crucial role in cellular diversity and function.
"Transcription is the first step that bridges the blueprint of DNA with the functionality of proteins, making its understanding imperative for advances in genetic research."
In summary, transcription may be viewed as a cornerstone of molecular biology, laying the groundwork for the synthesis of proteins that perform countless functions in a living organism. Understanding its complexities allows for deeper insights into genetic regulation, which can lead to advancements in gene therapy, biotechnology, and the treatment of genetic disorders.
Translation: The Synthesis of Proteins
Translation is a crucial phase in the protein synthesis mechanism, effectively bridging the gap between the genetic code embedded in DNA and the functional proteins that are essential for life. This process takes place in ribosomes, which are often described as the factories of the cell. The significance of translation cannot be understated, as it enables the cell to interpret the information carried by messenger RNA (mRNA) and synthesize the proteins needed to perform various biological functions.
From mRNA to Amino Acids
The journey from mRNA to amino acids is a fascinating sequence of events. Once transcription has produced an mRNA strand from DNA, this molecule exits the nucleus and enters the cytoplasm where ribosomes await. mRNA carries the genetic instructions written in the form of codons, which are groups of three nucleotides. Each codon corresponds to a specific amino acid, the building blocks of proteins.
Here’s a simplified breakdown of how this process works:
- Codon Recognition: Ribosomes scan the mRNA strand to find the start codon, usually AUG, which codes for methionine, the first amino acid in the synthesized protein.
- tRNA’s Role: Transfer RNA (tRNA) brings the appropriate amino acids to the ribosome, pairing with the codons on the mRNA strand through their anticodon region.
- Polypeptide Chain Formation: As each amino acid is added, a peptide bond forms between them. This elongation continues until a stop codon is reached, signaling the completion of the protein.
It is this sequence of recognition and bonding that allows cells to translate genetic code into essential proteins. The accuracy of codon-anticodon pairing is vital, as any misstep can lead to dysfunctional proteins, which might severely impact cellular function.
The Ribosome's Function
Ribosomes play an integral role in the translation process. They are composed of ribosomal RNA (rRNA) and proteins, functioning as both the site of translation and the catalyst for peptide bond formation. Understanding how ribosomes operate is fundamental to grasping the translation process.
The ribosome has three main sites essential for translation:
- A-site (Aminoacyl site): This is where the incoming tRNA carrying an amino acid binds to the mRNA.
- P-site (Peptidyl site): Here, the growing polypeptide chain is held and extended as new amino acids are added.
- E-site (Exit site): Once a tRNA has transferred its amino acid, it exits the ribosome from this site.
Ratios of activity and efficiency are paramount within ribosomes. The process of translation can be remarkably swift, with ribosomes capable of adding roughly 2-20 amino acids per second, depending on species and cellular conditions.
“Ribosomes do not only read the code; they are interpreting life’s blueprint, one amino acid at a time.”
Understanding the intricate interplay between mRNA, tRNA, and ribosomes not only sheds light on the synthesis of proteins but also highlights the complexity of cellular machinery. The efficiency of translation has profound implications in fields such as biotechnology, where synthetic biology aims to engineer proteins that could lead to trailblazing discoveries in medicine and industry.
Understanding Codons and Anticodons
In the grand narrative of molecular biology, codons and anticodons play leading roles when it comes to translating genetic information into functional proteins. These two terms, often tossed around in academic circles, represent more than mere jargon; they embody the elegance of genetic code. Codons, three-nucleotide sequences found in mRNA, dictate the amino acids that compose proteins. Anticodons, located on tRNA, are their complementary counterparts. Understanding these components is critical because they bridge the gap between DNA and the biological functions that sustain life.
This section will dissect the structure and function of codons, followed by a look at the significant role of tRNA. A key focus will be on how mutations in these sequences can disrupt protein synthesis, leading to various biological consequences.
Structure and Function of Codons
Codons are crucial elements of the genetic code. Each codon consists of three nucleotides, representing a specific amino acid. To put it simply, they are like words in a language, where each triplet provides a hint about what kind of protein will be created. The beauty of codons is their redundancy; multiple codons can specify the same amino acid. For instance, both UUU and UUC code for phenylalanine. This redundancy provides a buffer against mutation, a remarkable characteristic when considering the unpredictability of genetic alterations over time.
- Codons and their significance:
- Each of the 64 possible codons designates one of the 20 amino acids or serves as a stop signal during protein synthesis.
- The start codon, AUG, is particularly vital as it initiates the translation process, ensuring that the correct reading frame is established.
Understanding the structure and roles of codons also opens a door to the implications of coding efficiency. Certain codons are utilized more frequently, perhaps suggesting evolutionary advantages in adapting to specific environments.
The Role of tRNA
Transfer RNA, or tRNA, holds a unique position within the cellular machinery, acting as the interpreter in the world of codons. Each tRNA molecule is designed to recognize a specific codon on the mRNA strand and deliver the corresponding amino acid to the growing polypeptide chain. It’s almost like a delivery service that ensures the right ingredients are present while creating proteins.
A defining feature of tRNA is its three-dimensional structure that allows it to fold in a way that facilitates binding to its specific amino acid. The anticodon region of the tRNA molecule pairs with the complementary codon on the mRNA, ensuring that the correct amino acid is inserted in line with the genetic instructions. This pairing is not just about physical compatibility; it’s also about the precision of protein synthesis.
In summary, codons and anticodons function in concert to form a biological symphony. Any discord, such as mutations or mispairing, can result in defective proteins, which may lead to diseases or other biological dysfunctions. The understanding of how these elements operate is fundamental to advancements in molecular biology and genetics.
"In the world of biology, codons and anticodons represent the very language of life, encoding the instructions necessary for the creation of proteins that perform crucial functions in living organisms."
Additionally, the study of tRNA can lead to fascinating biotechnological applications, such as developing methods to combat diseases caused by faulty protein synthesis. To explore further on this topic, one might find insightful resources at Wikipedia or Britannica.
Through grasping the function and structure of codons and tRNA, we illuminate significant pathways in genetic translation. This knowledge not only enhances our understanding of life's fundamental mechanisms but also paves the way for future innovations in genetics and medicine.
Translation Process
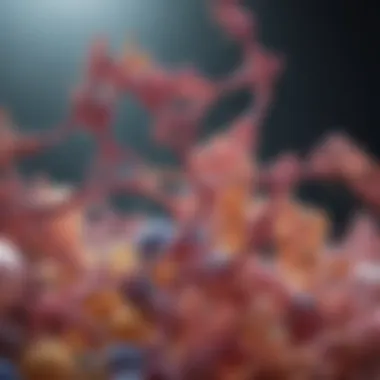
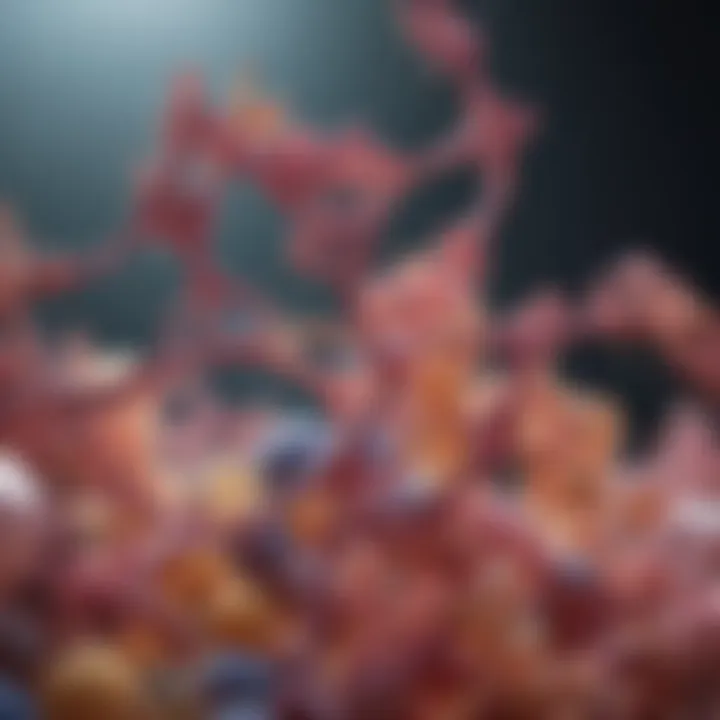
Translation is the critical second phase of protein synthesis, acting as the bridge that connects the genetic information encoded in DNA, via messenger RNA (mRNA), to the creation of functional proteins. This process is fundamental to all living organisms, effectively shaping everything from cellular structure to metabolic pathways. The importance of the translation process can't be overstated; it not only translates the language of nucleic acids into amino acids but also enables cells to produce the vast array of proteins necessary for survival and function.
Initiation, Elongation, and Termination
The translation process begins with three main stages: initiation, elongation, and termination. Each stage is intricately designed and relies on a variety of molecular players to ensure the proper synthesis of proteins.
Initiation
Initiation kickstarts translation. It involves assembling the ribosome, mRNA, and the first tRNA molecule, which carries the relevant amino acid. The ribosome scans the mRNA until it finds the start codon, typically AUG. This stage is crucial because any errors in this assembly can lead to faulty proteins, which might have severe consequences for the cell.
Elongation
Once the initiation complex is formed, the ribosome enters the elongation phase, which is where the bulk of polypeptide chain synthesis occurs. During elongation, the ribosome moves along the mRNA, matching each codon with the corresponding tRNA anticodon. As each tRNA molecule enters, the ribosome facilitates the formation of peptide bonds, linking amino acids together to form a growing polypeptide chain. This phase exemplifies the precision of the translation machinery, showcasing how the ribosome acts almost like a factory, tirelessly adding amino acids one after another.
Termination
Finally, termination occurs when the ribosome reaches a stop codon on the mRNA. This stage results in the complete polypeptide being released. Specialized proteins called release factors recognize the stop codon, prompting the disassembly of the ribosome complex. The completed protein may then fold into its correct three-dimensional shape, an essential step for functionality.
“The translation process not only constructs proteins but also reflects the beautiful complexity of life at a molecular level.”
In summary, the steps of initiation, elongation, and termination are not just a sequence of events but a highly coordinated and critical operation that ultimately determines the fate of the cell.
Post-Translational Modifications
After a protein is synthesized, it often undergoes various post-translational modifications (PTMs) that are essential for its final functionality. PTMs can fundamentally alter a protein's properties, affecting its activity, stability, and localization in the cell. Here are some key examples of post-translational modifications:
- Phosphorylation: Involves the addition of phosphate groups to amino acids like serine, threonine, or tyrosine, and plays a critical role in regulating the activity of enzymes and signaling proteins.
- Glycosylation: The addition of sugar molecules to proteins, which can influence protein folding, stability, and cellular recognition.
- Ubiquitination: Marks proteins for degradation when they are no longer needed or are damaged, thus maintaining cellular homeostasis.
These modifications highlight the dynamic nature of proteins—what starts as a simple polypeptide chain can transform into a complex functional entity through such changes.
Understanding translation, including its detailed steps and subsequent modifications, is crucial for multiple fields, including genetics, molecular biology, and biochemistry. This knowledge aids in developing therapeutic strategies, particularly in areas like genetic disorders and protein misfolding diseases.
Mutations and Their Impact
Mutations play a pivotal role in the world of molecular biology, acting as one of the primary forces behind genetic variation. Understanding mutations is crucial to grasping the broader scope of how genetic information translates into functional proteins. They can lead to various outcomes ranging from benign effects to profound changes in an organism’s biology. This section dives deep into different types of mutations—substitution, insertion, and deletion—and outlines their potential effects on protein synthesis. This understanding is not just an academic exercise; it has practical implications in fields such as medicine and biotechnology.
Types of Mutations
Substitution Mutations
Substitution mutations involve the replacement of one nucleotide with another. This seemingly minor alteration can have significant repercussions depending on where it occurs in the DNA sequence. One of the most compelling aspects of substitution mutations is their ability to either preserve the protein's function or disrupt it altogether. For instance, a common substitution mutation is the change of one amino acid in a protein—such as changing a valine to glutamic acid—which might lead to conditions like sickle cell disease.
- Key Characteristic:
- Unique Feature:
- Substitution mutations are often favored for their ability to introduce alernative protein variants without large-scale disruption of the genetic code.
- These mutations can be silent, missense, or nonsense, each having different implications for the final protein product.
The potential for both functional and dysfunctional outcomes makes substitution mutations particularly significant in this article, shining a light on their relevance in hereditary diseases and evolutionary biology. They serve as a reminder that even small changes can ripple through the genetic fabric, leading to vast ramifications.
Insertion Mutations
Insertion mutations occur when extra nucleotides are added into the DNA sequence. These mutations can shift the reading frame during the protein synthesis process, often leading to completely altered proteins. This type of mutation might seem like a bad actor on the genetic stage, with the potential for catastrophic results.
- Key Characteristic:
- Unique Feature:
- They can increase protein size or add extra domains, which can offer new functions or create dysfunctional proteins.
- Insertion mutations can be beneficial in some contexts; for example, they may enhance the adaptability of a species by introducing new traits.
However, the risk of generating nonfunctional proteins poses considerable challenges. Insertions often lead to significant health issues, and one prominent example is Huntington’s disease, caused by an insertion repetition in the HTT gene. Such instances highlight the dual nature of insertion mutations as both evolutionary tools and sources of disease.
Deletion Mutations
Deletion mutations entail the loss of nucleotides from the DNA sequence. Just like insertion mutations, they can dramatically alter the reading frame, resulting in proteins that might be completely dysfunctional or truncated. Their potential for harm is particularly noteworthy.
- Key Characteristic:
- Unique Feature:
- Deletions can cause various genetic disorders, one being cystic fibrosis, where a deletion in the CFTR gene leads to substantial physiological consequences.
- However, some deletion mutations can also result in advantageous traits in certain environments. For example, in bacterial populations, deletions can lead to antibiotic resistance.
The capacity of deletion mutations to produce significant disease offers a sobering view of genetic alterations. Their impact on protein synthesis exemplifies the delicate balance that governs mutation, efficacy, and health.
Effects on Protein Synthesis
Understanding the effects of mutations on protein synthesis provides a clearer picture of the potential results. Mutations can have wide-ranging impacts on how proteins are made, which ultimately dictates an organism's ability to function and survive.
"Mutations can serve as the building blocks of evolution but also embody the risk of catastrophic failure in protein systems."
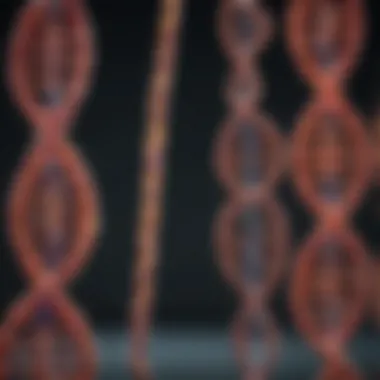
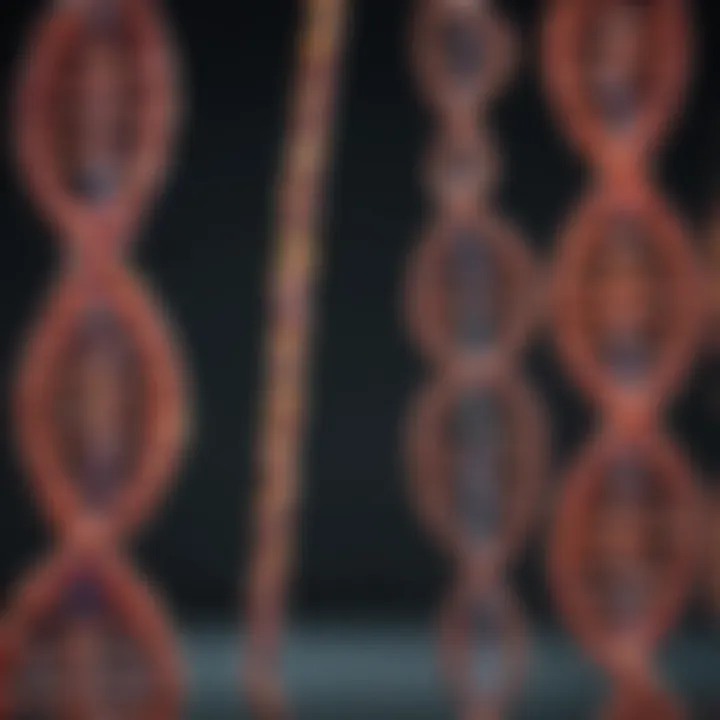
The interplay between these mutations and the steps of transcription and translation is instrumental.
- Substitution can lead to subtle but significant alterations in function.
- Insertion mutations may result in longer, oftentimes not functional proteins.
- Deletion mutations typically truncate proteins, which can be devastating depending on the critical roles those proteins play.
In summation, navigating the terrain of mutations requires an exceptional understanding of the implications for protein synthesis. Each mutation type carries its distinct flavor of consequences, underscoring the significance of molecular changes in the broader biological landscape.
Biotechnological Applications
The integration of molecular biology with technology has revolutionized various fields of science and medicine. At the crux of this evolution lies the understanding of protein synthesis, which fundamentally connects DNA sequences to amino acids. This relationship extends beyond mere theoretical knowledge; it has tangible impacts on industries ranging from agriculture to healthcare.
In the biological context, advancements in biotechnology rely heavily on manipulations made at the genetic level. By grasping how DNA encodes proteins, scientists can develop techniques that improve crop resistance, enhance livestock traits, and create innovative treatments for genetic disorders. These applications shape not only biomedical research but also agricultural practices, making the importance of understanding protein synthesis increasingly evident.
Furthermore, these applications are moving at a blistering pace. The implementation of biotechnology is leading to more efficient production methods for essential proteins and enzymes. Consequently, the implications of these biotechnological developments are both vast and promising, paving the way for novel therapeutics and improved agricultural resilience.
"Understanding the connection between DNA and protein synthesis can unlock new ways to address global challenges like disease and food security."
Genetic Engineering Techniques
Genetic engineering sits at the forefront of biotechnological applications, driving changes with the potential to reshape life itself. This multidisciplinary approach enables scientists to modify the genetic material of organisms to achieve specific outcomes. Techniques such as CRISPR (Clustered Regularly Interspaced Short Palindromic Repeats) illustrate this evolution. CRISPR allows researchers to cut and edit genes with high precision. As a result, it helps in constructing genetically modified organisms (GMOs), which can thrive in harsh conditions or have enhanced nutritional values.
Some commonly used genetic engineering techniques include:
- Recombinant DNA technology: This involves combining DNA from different sources, allowing for the production of proteins that wouldn't otherwise occur in nature.
- Gene cloning: By creating copies of specific genes, this approach facilitates the study of gene function and expression.
- Gene therapy: This technique aims to treat or prevent diseases by directly altering the genes responsible for the conditions.
These methods are not merely academic; they offer profound implications for public health, crop improvement, and even biofuels. Each technique provides robust tools that are reshaping our understanding of life’s processes while directly addressing pressing needs in society.
Protein Production and Therapeutics
The demand for therapeutic proteins has skyrocketed over the last decades, making protein production a crucial aspect of biotechnological applications. These proteins often form the basis for crucial medicines, vaccines, and various health supplements. Utilizing genetically modified organisms, especially bacteria and yeast, allows for high-volume production of these proteins safely and efficiently.
A few noteworthy examples include:
- Insulin: Initially extracted from animals, insulin is now produced through recombinant DNA technology, significantly increasing its availability for diabetes patients.
- Monoclonal antibodies: These proteins are engineered to target specific antigens, providing precise treatments for conditions like cancer and autoimmune disorders.
- Enzyme replacement therapies: For genetic diseases where specific enzymes are lacking, engineered proteins can replace them, dramatically improving patients’ quality of life.
Protein production through biotechnological methods is not just a scientific phenomenon; it’s a societal imperative. The therapeutic implications of these proteins correlate directly with enhanced patient outcomes and can even result in cost-effective healthcare solutions.
As we move forward, the fusion of biotechnology and molecular biology will likely spur innovations that resonate within the healthcare landscape, offering hope in areas that have long faced treatment challenges.
Therapeutic Implications
The confluence of genetic understanding and therapeutic application has transformed biotechnology and medicine in recent years. This section delves into the significance of therapeutic implications, revealing how the connection between DNA and amino acids fosters innovations that can change lives. The intricate processes of gene therapy and protein-based drugs hold the potential to alter the landscape of treatments, paving the way for personalized medicine.
Gene Therapy
Gene therapy emerges as a revolutionary approach, aiming to correct or replace faulty genes responsible for various diseases. The premise is straightforward yet profound—introducing functional genes into a patient’s cells can alleviate or even cure ailments that once seemed insurmountable. For instance, patients with inherited conditions like cystic fibrosis or hemophilia benefit significantly from gene therapy. In cystic fibrosis, where a single malfunctioning gene leads to severe respiratory issues, replacing that gene can change the course of the disease.
The circulating narratives around gene therapy are as optimistic as they are complex. Consider the challenges: delivery mechanisms must navigate through a patient’s circulatory system, and once at the target site, the therapy must integrate seamlessly with the host's genetic structure. Techniques like viral vectors—where modified viruses carry therapeutic genes—have proven beneficial, bridging a gap that therapeutic interventions could not quite cover before.
"Gene therapy has the potential to address the root cause of diseases rather than merely treating symptoms."
Protein-Based Drugs
In addition to gene therapy, protein-based drugs represent another pinnacle of modern therapeutics. These medications are designed to mimic or enhance the action of natural proteins in the body, resulting in innovative therapies for conditions ranging from autoimmune disorders to certain cancers. For example, monoclonal antibodies have become a cornerstone of cancer treatment, targeting specific cells with high precision while minimizing damage to healthy tissues.
One remarkable aspect of protein drugs is their tailored nature. They can be engineered using recombinant DNA technology, allowing for customization to fit specific patient needs. This bespoke approach elevates treatment efficacy and significantly reduces potential side effects, showing that one size does not fit all in modern medicine.
The application of protein-based drugs can also reflect evolutionary principles, as nature has perfected various proteins over millennia. Harnessing these proteins involves delicate balancing acts—ensuring stability, bioavailability, and activity throughout treatment.
Through these avenues—gene therapy and protein-based pharmaceuticals—the therapeutic implications of the DNA to amino acids journey illustrate a bright horizon in medical innovation. As science continues to advance, the goal of transforming lives becomes ever more attainable, urging ongoing research and ethical consideration in this burgeoning field.
End
The significance of the journey from DNA to amino acids cannot be overstated. This article has traversed through the complex mechanisms of molecular biology, presenting a detailed view of how genetic information is translated into functional proteins. At its core, understanding this intricate relationship is crucial not just for students and researchers in the field but also for scientists involved in various practical applications such as genetic engineering and therapeutic development.
In breaking down the essential processes, we highlighted the role of transcription and translation, the importance of codons, and the impact of mutations. Each element plays an indispensable part in the tapestry of life, dictating everything from basic cellular function to advanced biotechnological innovations. Furthermore, the exploration of therapeutic implications opens new avenues for improving human health, showcasing how foundational knowledge can lead to transformative advancements.
Key takeaways from this exploration include:
- The clarity of how DNA encodes the necessary instructions for protein synthesis.
- An understanding of the indispensable roles played by RNA during both transcription and translation processes.
- Insight into the repercussions of mutations, which can alter the course of protein synthesis and lead to numerous diseases, emphasizing the need for vigilant genetic research.
As we have discussed, comprehending these mechanisms empowers not only further academic inquiry but also practical applications in medicine and biotechnology, thus enhancing the quality of human life.
Summarizing Key Points
Throughout this article, we navigated through several key points that underscore the importance of understanding the DNA-to-amino-acid journey. Here are the highlights:
- Structure and Function: The structure of DNA serves as the blueprint for life, with nucleotides forming the basis of genetic code.
- Transcription and Translation: These two crucial processes transform the genetic information stored in DNA into functional proteins, illustrating the transition from nucleotides to amino acids.
- Mutations: Not all mutations are detrimental; some can lead to advantageous traits, while others may cause diseases, reflecting the dual nature of genetic variation.
- Biotechnology Applications: Genetic engineering leverages insights from molecular biology to create innovations in medicine, agriculture, and industry.
The synthesis of this knowledge not only enriches our scientific discourse but also carries ethical considerations regarding its application, further highlighting the need for responsible research.
The Future of Genetic Research
Looking ahead, the future of genetic research holds immense potential. With advancements in technologies such as CRISPR-Cas9 and other gene-editing tools, scientists are now equipped to make precise modifications to the genetic code, raising exciting prospects.
- Precision Medicine: As genetic research continues to evolve, so will the methods for tailoring medical treatments based on individual genetic profiles. This aims to enhance effectiveness while minimizing side effects.
- Synthetic Biology: The merging of biology with engineering opens doors to the creation of entirely new biological parts, systems, or organisms, providing powerful tools for solving complex challenges in health and environmental fields.
- Ethics and Regulation: With these advances come significant ethical considerations. Discussions around gene editing and its implications for society are paramount to ensure responsible stewardship of genetic technologies.